The sequencing of the human genome at the turn of the 21st century was heralded as a monumental scientific achievement. Scientists around the globe expected that this new-found technology would accelerate understanding of human biology and revolutionize medicine by identifying genetic causes of disease, which could then be cured with gene therapy
From an environmental health perspective, it was believed that these advances would allow us to understand how our personal genetic background influenced our susceptibility to environmental exposures, and this information would enable preventative strategies tailored to an individual’s genetic predispositions. However, as genomic studies advanced, a perplexing issue emerged: while numerous genetic variants were identified among human populations, their ability to predict common diseases was surprisingly limited [1]. In many cases, these variants explained only a small fraction of disease risk, leaving a substantial portion unexplained – a phenomenon known as “missing heritability.” This unexpected reality of the genetic revolution prompted a shift toward exploring other mechanisms of inheritance that explained how the environment interacted with the genetic code to influence individual susceptibility to health and the transmission of altered health effects.
While DNA is the genetic blueprint that encodes the information necessary for all life on Earth, carrying out the instructions of the genetic code is not solely determined by the DNA sequence but is also influenced by an additional layer of regulation that dictates when and how genes are turned off or on. These additional regulatory mechanisms for controlling gene function are termed ‘epi’-genetics, which literally means “above the gene”. More specifically, epigenetics regulates the structure and function of DNA independent of changes in the genetic sequence [2].
As illustrated in Figure 1, the central dogma of biology describes the flow of information from DNA to RNA to protein. This view of biological information transfer is now regarded as an oversimplification. Epigenetic factors introduce a layer of control by regulating the flow of genetic information in response to environmental cues. One way to think about this is that the genetic code represents the printed words in the recipe book of life, while epigenetics are the bookmarks, highlights, and notes that determine how those words are read and interpreted. By regulating gene expression without altering the genetic sequence, epigenetics acts as a mediator between the environment and the expression of our DNA.
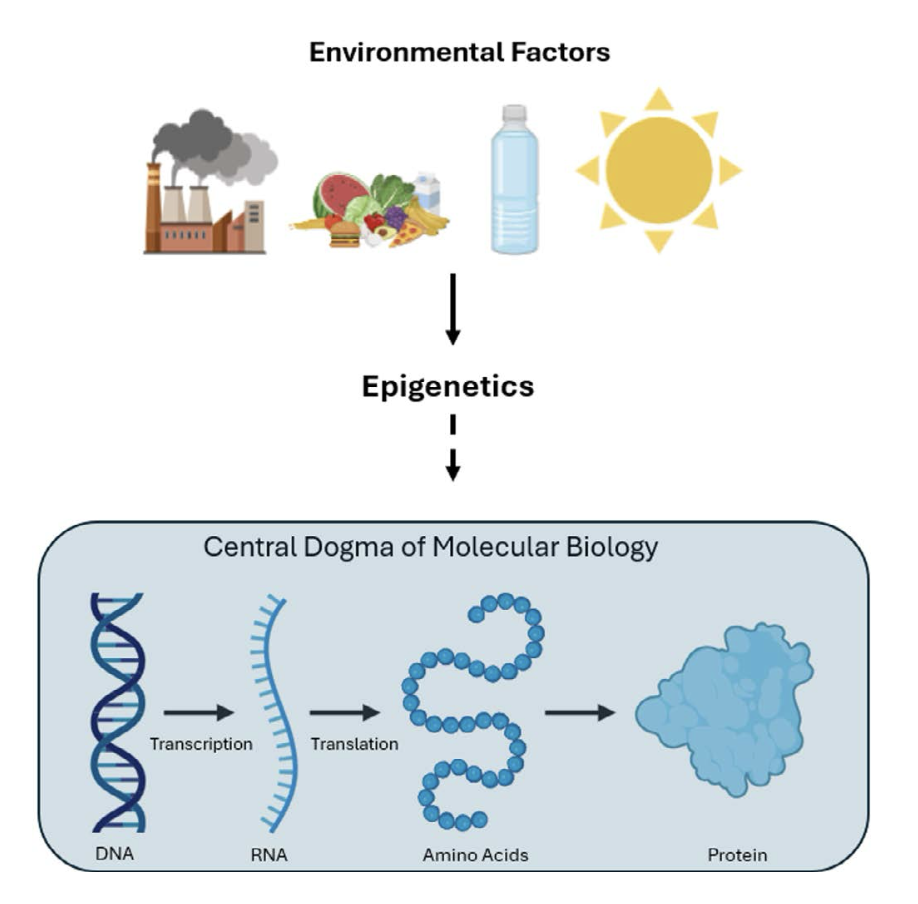
Unlike DNA, which is relatively stable within an individual, epigenetic changes are dynamic. This plasticity is essential for survival, enabling individuals to adapt to changing environments within the limits of their genetic code. Yet, this adaptability also presents risks. For example, exposures to chemical contaminants in the environment can alter epigenetic signals, leading to dysregulation of gene expression in ways that can negatively impact human health. Some of these disease promoting changes in the epigenome can be passed down to unexposed progeny – a concept known as transgenerational inheritance (TGI). In today’s increasingly polluted world, where our cells and the cells of our children are under constant assault from chemical hazards, such as endocrine disrupting chemicals (EDCs), heavy metals, and an ever-growing list of emerging environmental contaminants, the potential for these exposures and other environmental stressors (e.g., noise pollution, temperature extremes, nutritional deficiencies, etc.) to induce harmful epigenetic changes is a growing concern. As we continue to grapple with the implications of TGI, it becomes clear that understanding the complex interplay between genetics, epigenetics, and environmental stressors is crucial for developing targeted interventions for mitigating the adverse health impacts of these exposures on not only the exposed individual, but also future generations.
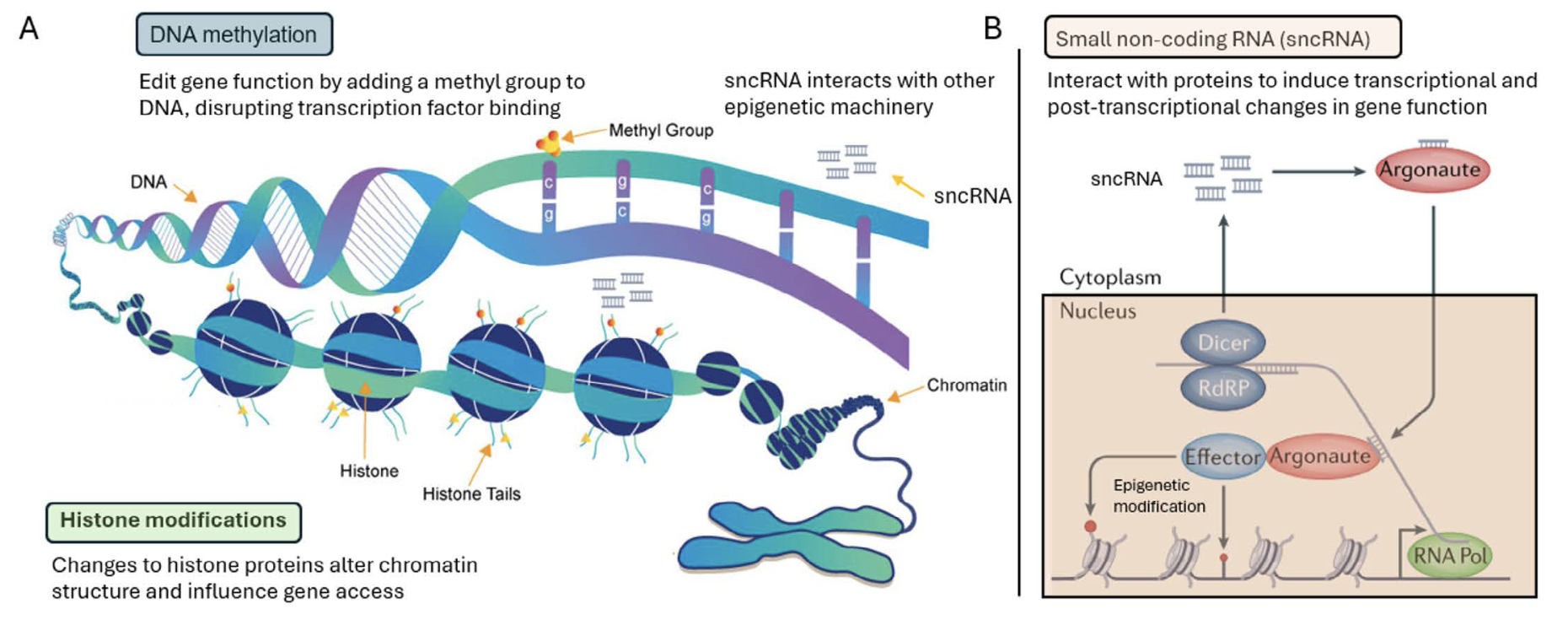
Mechanisms of epigenetic inheritance
Epigenetic inheritance explains how environmental factors influence gene expression within and across generations. A central mechanism for regulating gene expression is DNA methylation (Figure 2A), one of the most extensively studied types of epigenetic modifications. DNA methylation involves the addition of a methyl group to the cytosine base of CpG dinucleotides within DNA, forming 5-methylcytosine (5mC). This modification typically acts as a repressive mark that prevents the binding of transcription factors, thereby turning ‘off’ gene expression. Unlike the genetic sequence, DNA methylation can be readily altered, with frequent modifications occurring throughout our lifespan. Enzymes from the Ten-Eleven Translocation (TET) family can oxidize 5mC to 5-hydroxymethylcytosine (5hmC), which is generally associated with active transcription, thereby turning ‘on’ genes. This process highlights the dual nature of DNA methylation – it is capable of both repressing and permitting gene expression depending on the environmental context and DNA modifications involved. Moreover, DNA methylation patterns are heritable in vertebrates, being faithfully transmitted and preserved through cell division by maintenance methyltransferases such as DNMT1. These cellular mechanisms enable the inheritance of altered methylation, or methylation marks, induced by environmental exposures to be passed down through the germline, ultimately influencing offspring health independent of genetic changes.
Histone modifications represent another mechanism of epigenetic regulation (Figure 2A). Histones, the proteins around which DNA is wrapped to form nucleosomes, undergo various post-translational modifications, including acetylation, methylation, phosphorylation, and ubiquitination. These modifications occur primarily on the lysine-rich amino-terminal tails of histones and play a critical role in determining the structural dynamics of DNA. For instance, lysine acetylation generally results in a more relaxed chromatin structure, facilitating gene transcription, whereas methylation can either activate or repress transcription depending on the specific lysine residue that is modified. Notably, these histone marks can be modified by environmental exposures and inherited. Modified histones are re-incorporated into newly synthesized DNA during replication, ensuring that epigenetic information in the form of chromatin structure is preserved and propagated. The role of histone modifications in TGI is particularly intriguing. For example, trimethylation of lysine 9 on histone H3 (H3K9me3) is a key mark associated with the formation of heterochromatin, which condenses or ‘silences’ surrounding DNA. This mark is maintained by the action of histone-specific methyltransferases, along with the recruitment of other regulatory proteins that promote propagation of this repressive state across generations. These modifications illustrate how the chromatin landscape can be molded by environmental exposures, with the potential for these changes to be inherited across multiple generations.
Chromatin structure is not uniform; it varies between tightly packed heterochromatin and more loosely packed euchromatin. The three-dimensional state of the chromatin within the nucleus, often referred to as nuclear architecture, affects gene accessibility to transcriptional machinery and thus, plays a critical role in gene regulation and epigenetic inheritance. Environmental exposures can influence gene expression by inducing changes in the nuclear architecture. For example, hypermethylation of DNA is typically associated with the formation of heterochromatin, leading to gene silencing, while hypomethylation is linked to euchromatin and active transcription. These structural changes in chromatin can be preserved across cell divisions and potentially transmitted through the germline, contributing to the inheritance of epigenetic states and associated phenotypic traits.
Small non-coding RNAs (sncRNAs), which include microRNAs (miRNAs), small interfering RNAs (siRNAs), and PIWI-interacting RNAs (piRNAs), comprise yet another layer of epigenetic regulation. These small molecules are involved in fine-tuning gene function at both transcriptional and post-transcriptional levels. For instance, miRNAs and siRNAs are best known for their ability to cause targeted mRNA degradation or translational repression. They achieve this by first being modified into an Argonaute complex (Figure 2B). Once formed, this complex binds to complementary sequences on target mRNAs, leading to either cleavage and degradation of the mRNA (a process typically associated with siRNAs) or inhibits translation (more common for miRNAs), depending on the level of complementarity between the sncRNA and the target mRNA. In addition, Argonaute complexes can recruit other epigenetic modifiers to specific positions within the genome, facilitating DNA methylation of specific DNA sequences or acetylation of specific histones.
In the context of TGI, piRNAs are particularly impactful due to their critical role in silencing transposable elements (a nucleic acid sequence in DNA that can change its position within a genome, sometimes creating or reversing mutations and altering the cell’s genetic identity and genome size) within the germline. By guiding PIWI proteins to degrade transposon RNA, piRNAs help maintain genomic integrity. Additionally, piRNAs can establish repressive chromatin states that prevent transposon activity, further protecting the genome across generations. The presence of various sncRNAs in germ cells, especially sperm, suggests their involvement in the transmission of epigenetic information from one generation to the next.
In summary, the mechanisms of epigenetic inheritance—including DNA methylation, histone modifications, sncRNAs, and overall nuclear architecture—form a regulatory network that controls gene expression. While these processes are often examined separately, it is important to recognize that these mechanisms interact to guide the interpretation of our genetic instructions. These mechanisms are not only crucial for normal development and cellular differentiation, but also provide a means by which environmental factors can cause lasting changes in the expression of the genome. Through the processes of mitosis and meiosis, these epigenetic marks can be passed down to future generations, leading to TGI. Understanding these mechanisms is essential for unraveling how environmental exposures influence health across generations [3].
Passing down epigenetic information: Copying and rebuilding through generations
The acquisition, maintenance, and transmission of epigenetic information to daughter cells generated by mitosis and meiosis might suggest that environmentally induced changes in the epigenome would be permanent. However, in higher eukaryotes, particularly vertebrates and mammals, genome-wide reprogramming events that occur during gametogenesis and early embryogenesis can erase methylation marks and reset histone modifications. Reprogramming is a critical component of vertebrate and mammalian development because it enables pluripotency. Thus, changes in the epigenome induced in an individual do not necessarily persist in subsequent generations. Because of this natural barrier to the inheritance of epigenetic information, it is believed that the transmission of epigenetic marks across generations occurs through both replicative and reconstructive mechanisms. Replicative transmission involves the faithful copying of DNA methylation and histone modifications during cell division: methylation patterns are recognized and re-established post- replication, and modified histones are distributed between daughter strands. As a result, epigenetic states are maintained through self-reinforcing loops. This process has been observed in organisms like yeast, where epigenetic marks are maintained through meiosis. Some regions in the DNA, such as imprinting control regions, demonstrate resistance to reprogramming, suggesting that replicative mechanisms may occur in specific contexts. Reconstructive mechanisms, on the other hand, suggest that even when epigenetic marks are erased, they can be faithfully reconstructed based on secondary signals. This process is hypothesized to involve non-coding RNAs or other signals that orchestrate the restoration of the parental epigenetic pattern. For example, non-coding RNAs transmitted through sperm can influence DNA methylation and histone modifications in the developing embryo even when the original DNA methylation and histone marks have been removed, effectively reconstructing the original epigenetic landscape [3]. While these dual mechanisms of replicative and reconstructive transmission highlight the resilience and adaptability of epigenetic information, it is important to note that demonstrating these processes in vertebrate model systems remains a significant challenge, underscoring a gap in our knowledge regarding how epigenetic marks are inherited. This gap represents a critical area for future research since uncovering these mechanisms could provide insights into how marks are inherited in humans.
The critical role of epigenetics in development and disease
Epigenetic changes are not just a consequence of environmental exposures but are fundamental to the normal development and maintenance of cellular identity, ensuring proper gene expression throughout life. From the earliest stages of development, these mechanisms play a critical role in guiding the differentiation of cells into the specialized tissues and organs that make up the human body via differential gene expression in different cells and tissues at very specific times in development. This process begins immediately following fertilization, when the paternal genome undergoes rapid DNA demethylation and histone modification. This initial reprogramming is followed by a new wave of embryonic methylation that establishes the epigenetic blueprint for the developing embryo, a blueprint that must be meticulously maintained to ensure proper gene function and development into later life.
Disruptions to the carefully orchestrated patterns of epigenetic modifications in development can lead to a wide array of congenital disorders and multisystem pediatric syndromes. For example, genomic imprinting, a process regulated by DNA methylation, determines whether specific genes are expressed based on whether they are inherited from the mother or father. Errors in this process can lead to imprinting disorders such as Prader-Willi and Angelman syndromes, which result in severe developmental and neurological impairments [4].
In addition to congenital disorders, epigenetic abnormalities are increasingly recognized as significant factors in the development of chronic diseases such as cancer. For example, DNA methylation plays a dual role in cancer progression: hypomethylation can activate oncogenes, leading to chromosomal instability, while hypermethylation can silence tumor suppressor genes, contributing to uncontrolled cell growth and metastasis. These changes are common across various types of cancer, making them critical targets as diagnostic biomarkers and for therapeutic interventions. Similarly, epigenetic errors contribute to autoimmune disorders, where aberrant DNA methylation patterns can trigger inappropriate immune responses, leading to conditions such as lupus [4].
Epigenetic mechanisms are not static; they evolve with age, an observation that has given rise to the concept of an ‘epigenetic clock,’ which estimates biological aging by assessing specific DNA methylation patterns. As individuals age, the accumulation of altered methylation patterns and other epigenetic changes is thought to contribute to the onset of age-related diseases, including neurodegenerative diseases such as Alzheimer’s and Parkinson’s, cardiovascular conditions like atherosclerosis, and metabolic disorders such as type 2 diabetes. Age-related epigenetic alterations have also been implicated in various cancers, indicating that they may play a role in the development and progression of malignancies. The potential to reverse epigenetic aging is a promising area of research, with methods like partial reprogramming using ‘Yamanaka factors’ showing potential in rejuvenating tissues and reversing age-related biomarkers in experimental settings. These interventions aim to reset the epigenetic clock, restore youthful epigenetic profiles, and potentially mitigate the effects of aging. Recent studies indicate that such DNA methylation-based biomarkers could not only help identify the biological age of various tissues, but also serve as valuable tools for validating anti-aging therapies and understanding the fundamental mechanisms driving the aging process [5].
Proper epigenetic regulation is crucial for normal development and preventing a broad spectrum of diseases throughout life. The carefully orchestrated interaction between environmental factors, epigenetics and genetics is critical to human health, and perturbations of these interactions influence individual susceptibility to disease. The ongoing exploration of these mechanisms holds the potential to unlock new strategies for maintaining health of individuals and across generations.