Long before the existence of microbes was known, humans were unknowingly harnessing their power through practices such as brewing, bread leavening, or cheesemaking. These processes extended the shelf life of food, enhanced its nutritional value, and significantly contributed to the advancement of industry and civilization
At the heart of these practices lies the process of fermentation, where sugars are transformed by different microorganisms into acids or alcohols. Fermentation processes are anaerobic, requiring an environment devoid of oxygen for optimal performance. Initially unaware of the invisible microbial agents at work behind this process, humans gradually developed specialized containers designed to create and maintain the necessary growth conditions. However, humanity can no longer afford to build new reactors based on centuries of trial and error. Instead, we must focus on advancing technologies and establishing measurement techniques that can accelerate the pace of scientific discovery.
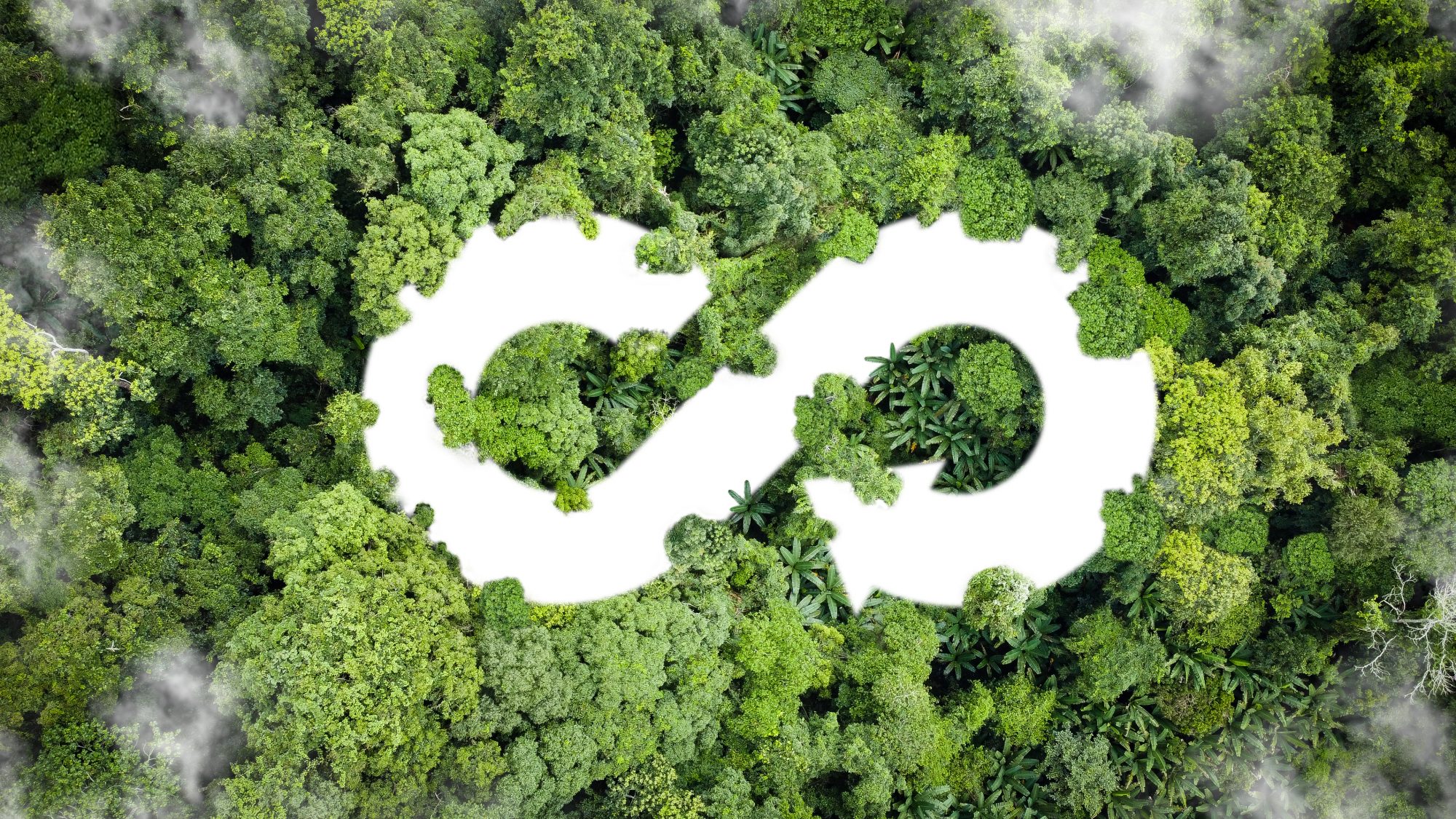
Modern microbial biotechnology requires specialized equipment
Fast forward to the present, fermentation containers have evolved into what we now call bioreactors and biosynthesis has become a cornerstone of our economy. With the advancement of our understanding of microbiology and cellular metabolism, today’s bioreactors are specially designed systems that make use of a spectrum of other microbial synthesis capabilities beyond fermentation. They are vital tools in biomanufacturing, enabling the large-scale production of a wide array of products, including biofuels, pharmaceuticals, enzymes, and specialty chemicals.
Moreover, bioreactors play a crucial role in maintaining optimal conditions for cellular growth and production. They ensure maximal yields by offering control over several environmental parameters such as temperature, pH, oxygen and other gas levels, and nutrient supply. However, as new microbial capabilities are steadily discovered, the need for methods that facilitate and accelerate the study and understanding of ideal growth and production conditions has become increasingly evident. Such advances are, therefore, crucial for developing sustainable solutions to current existential challenges faced by society.
Electroactive microbes can lead to sustainable plastic production
Oxidation-reduction reactions represent the foundation of energy generation in most life forms. These reactions involve the exchange of electrons between two chemical species. Microbes source the electrons needed for their metabolic processes from the environment via indirect or direct pathways. While soluble electron donors or acceptors are utilized by most microbes, some microbes have developed the ability to exchange electrons with insoluble solid materials through a process called extracellular electron transfer (EET).
EET interactions are bidirectional and can be categorized based on the direction of electron flow. Reductive EET takes place when bacteria reduce insoluble substances through respiration, with the electrons flowing from the bacteria to the solid substance. In contrast, extracellular electron uptake (EEU) is the opposite process in which insoluble substances are oxidized and electrons flow from the solid substance to the bacteria.
EEU is a relatively recent discovery and is one microbial process that can potentially be used for sustainable microbial synthesis. One focus of the Bose research group at Washington University in Saint Louis is exploring how we can leverage this capability by understanding the molecular mechanisms behind EEU.
The model EEU-capable organism studied by the Bose lab is Rhodopseudomonas palustris TIE-1. TIE-1 is a phototrophic organism that in a process like photosynthesis can use light as a primary energy source coupled with uptaking electrons from a solid substance to convert carbon dioxide into biomass. This process could not only help decrease environmental carbon dioxide, but can also simultaneously produce bioplastics or biofuels through microbial electrosynthesis (MES).
The Bose lab shows that EEU is a widespread microbial ability in nature that can be tied to organisms beyond phototrophs and permits microbial survival in environments where soluble electron donors are limiting. They have shown that EEU is present in marine anoxygenic bacteria and are exploring other EEU-capable microbes, such as Rhodomicrobium, that would be more efficient for MES. Thus, developing tools that can help us screen for MES-capable microbes and their preferred growth conditions faster becomes a necessary step in our work towards a circular economy and sustainable future.
Bacteria could help us develop new pharmaceuticals
While it may seem counterintuitive, bacteria are vital players in the pharmaceutical industry. The general public often attributes drug discovery to synthetic chemistry using molecules produced by lab scientists. However, nature is a long-recognized source of drugs, too, recalling the antibiotic penicillin produced by the penicillium mold. The reality is that microbially biosynthesized drugs are far more important to medicine than commonly acknowledged.
For natural drug production, filamentous actinomycete bacteria, Streptomyces and their close relatives in particular, are especially noteworthy. These microbes have a complex lifestyle more akin to fungi than most bacteria. They are found in a multitude of diverse environments, and have evolved to interact with and adapt to these surroundings, sometimes via chemical warfare against other microbes. Since the 1940s they have been pursued as key contributors to drug discovery. In fact, they are the source of two-thirds of clinically utilized antibiotics, as well as a variety of anticancer, antifungal, and immunosuppressive drugs.
The Blodgett lab at Washington University in Saint Louis is dedicated to uncovering the processes by which actinomycetes control drug-like molecule production. For bacteria to produce these secondary metabolites, they require specialized sets of biosynthetic genes organized into discreet clusters, a feature that opens the door to genomics-based molecule discovery.
To demonstrate how genome sequences can predict the existence of yet-undiscovered drugs, the Blodgett lab recently turned its attention to a rare actinomycete, Lentzea flaviverrucosa. This bacterium harbors a specific genetic marker indicating the potential to produce piperazate-containing molecules. Given that similar molecules have demonstrated cytotoxic properties against cancer cells in the past, pursuing Lentzea for new related molecules seemed particularly promising. In collaboration with the Cao Lab at the University of Hawaii Cancer Center, the Blodgett lab was able to identify two new bioactive molecules produced by L. flaviverrucosa that exhibited bioactivity against cultured cancer cells.
L. flaviverrucosa stands out in this story not only because of its medically-interesting chemistry but also because it demonstrates how rarer and less-studied bacteria can lead to valuable products. The idea that we can leverage diverse microbes for human benefit is timely and important, especially given the escalating antibiotic resistance crisis and the need for treating various diseases with no known cures. Thus, the significance of studying less common bacteria for drug discovery cannot be overstated. Who knows what new microbial product may be lurking in soils from oceans, deserts, swamps, caves; or even our own backyard?
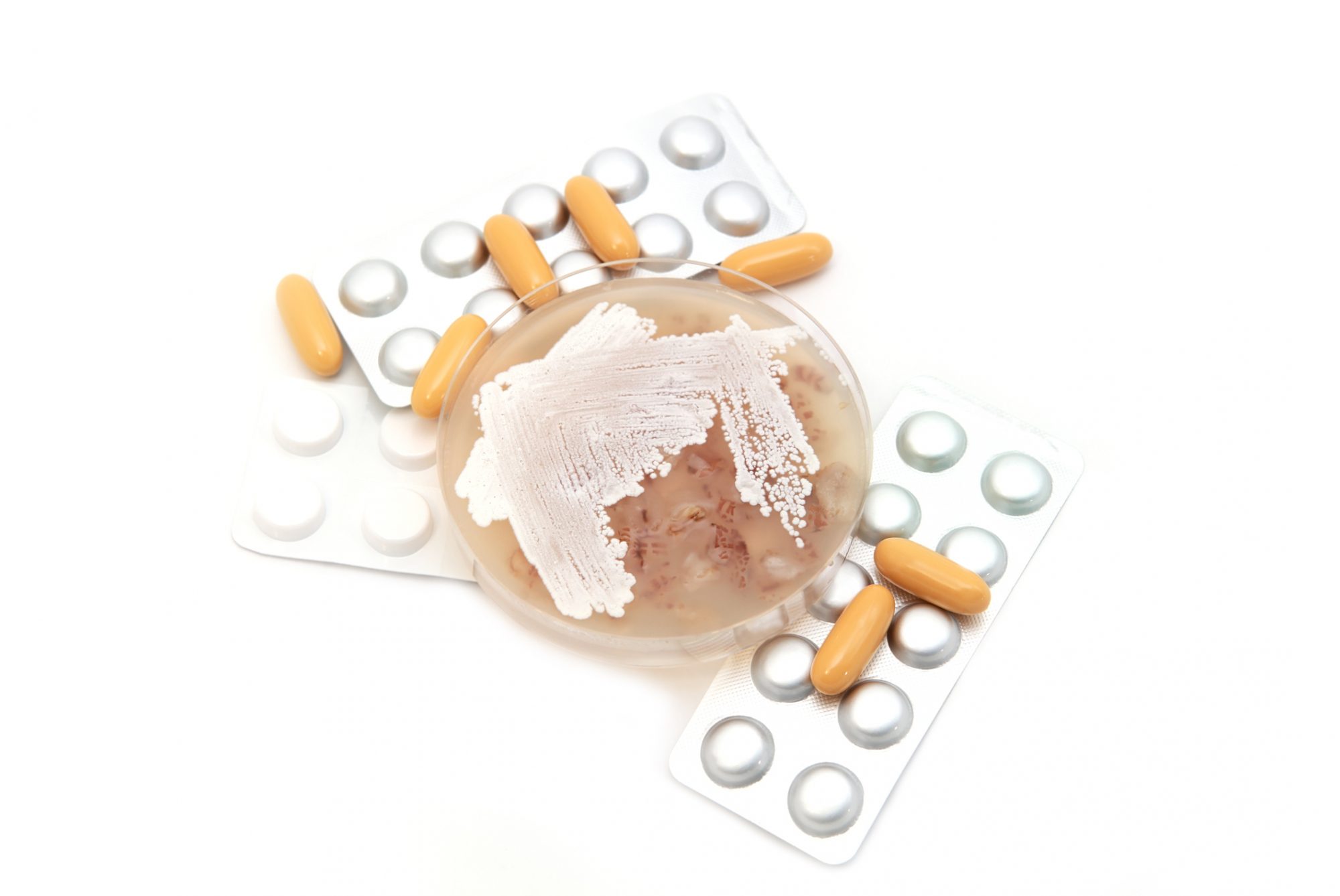
We need better laboratory tools to understand the fundamental processes behind microbial synthesis
Studying EEU-capable microbes and biologically active compound-producing bacteria in the laboratory poses its own unique challenges. While both microbes are prevalent in different natural habitats, from deep-water sediments in the case of some EEU-capable microbes to a wide range of soils for various Streptomyces and other Actinomycetes, recreating their natural environment in the lab can become impossible and likely impractical for optimized microbial synthesis and metabolite extraction.
EEU-capable microbes and electroactive bacteria require a source of electrons for growth and, subsequently, microbial electrosynthesis. In nature, this source is typically represented by solid minerals. However, in the laboratory the electron source is represented by poised electrodes inside of a larger bioelectrochemical system (BES) or bioelectrochemical reactor. These electrodes are maintained at various potential ranges, matching the potentials of materials that microbes oxidize in their natural environment, using potentiostats. The potentiostats not only provide the electron source at a stable potential, but also allow for tracking different electrochemical parameters in the system.
Like other bacteria, culturing Streptomyces and Actinomycetes can be accomplished in either stirred liquid or on solid agar within culture plates. The choice of media often depends on the ensuing experiments intended for the bacteria. These can range from wanting optimal production of secondary metabolites to compatibility with an array of downstream genetic manipulation procedures. To add to the complexity, the optimal growth conditions and media differ among species, necessitating the screening of various liquid and agar solutions.
Determining the optimal culturing conditions for microbes calls for cross-disciplinary solutions, incorporating insights from microbiology, genetics, chemistry, materials science, and engineering. To truly grasp these complex systems, we need innovative tools and methods that can integrate and measure the various aspects behind microbial synthesis. It’s important to note that while current macro-level methods used in the laboratory offer a broad view of these processes, a more in-depth understanding necessitates a microscale perspective. By delving into the microscale, we can explore these intricate interactions at a level of detail that is simply not possible with macro-scale approaches.
Miniaturization can allow us to study microbial synthesis at the microbial level and can expedite scientific progress
Through the miniaturization of our measurement instruments, we could manage, quantify, and observe microbial synthesis in a way that mirrors the size scale of the actual microbes. Devices known as lab-on-a-chip, which consolidate and integrate several lab processes on a handheld platform, have already considerably enhanced our comprehension of human health, along with other physical and chemical phenomena. These devices not only provide a method for combining different measurement systems, but also offer numerous advantages such as decreased sample volumes, in-situ analysis, expedited processing times, and superior measurement resolution.
In a collaborative effort, the Bose research group and the Meacham lab group are developing high-throughput microfluidic bioelectrochemical devices specifically designed for the exploration of microbial EEU. The microfluidic component of our devices simplifies the process of distinguishing between the behavior of microbial biofilms and planktonic cells. It also allows the use of a chemical probe approach in discerning how EEU is affected or inhibited by different chemical factors.
Additionally, the fusion of in-situ imaging techniques with electrochemical measurements holds the potential to augment our comprehension of biofilm formation. This is made possible by enabling the real-time observation of microbial biofilm growth, as opposed to limiting the observation to post-experiment analysis. We can do this by miniaturizing the electrochemical reactor and opting for transparent electrode materials such as indium tin oxide, that are both biocompatible and allow for in-situ imaging.
Miniaturized systems can enable high-throughput screening by allowing for the simultaneous testing of multiple electrode materials in the case of new EEU microbes or different culture media for new or rare bioactive compound-producing bacteria under identical conditions. This is possible because the smaller devices can incorporate numerous individual reaction chambers or channels, each of which can be paired with a different electrode material or culture media. This parallel approach significantly reduces the time required to identify promising candidates compared to sequential testing in larger-scale setups.
Microscale measurement and culturing systems can also offer more precise control over the physical and chemical environment, which can help isolate the effects of different parameters inside the bioreactors. This fine control can enhance the reliability of the screening process and provide clearer insights into the relationship between the physical and chemical variables and microbial synthesis and growth performance.
Additionally, microfluidic systems require smaller media volumes and fewer bacterial cells, reducing the cost and preparation time for each experiment. This efficiency can make it practical to test more conditions within a given budget or timeframe.
Moreover, miniaturization can potentially allow for studies at the single-cell level. Understanding the interaction of individual bacteria with different electrode materials or under different growth conditions could provide unprecedented insights into the mechanisms of microbial electrosynthesis. On a similar note, a single-cell PDMS-based microfluidic device was developed to study the germination and growth of Streptomyces lividans, a biologically active compound-producing bacteria.
However, scaling down bioreactors for the investigation of EEU-capable microbes and drug-producing bacteria presents a series of obstacles ranging from choosing biocompatible materials capable of sustaining conditions conducive to microbial growth to designing devices that allow for the incorporation of different in-situ measurement systems.
Bioreactor miniaturization represents a multidisciplinary problem-solving approach
Material choice and design is paramount in the construction of the miniaturized bioreactor as it dictates the downstream processes that can be achieved with the miniaturized device. The materials need to be biocompatible, chemically stable, and able to withstand the pH of the growth media, as well as the pressure and temperature conditions necessary for bacterial growth.
Specific to EEU-capable bacteria, the miniaturized electrodes not only need to be biocompatible but also capable of withstanding the growth conditions for several days without corroding. This remains a particular challenge, especially in developing reliable miniaturized reference electrodes for accurate potential measurements.
Maintaining sterility during the setup and operation of the miniaturized bioreactors can be challenging. The devices must be fabricated and assembled in a way that allows them to be sterilized before bacterial inoculation. Additionally, preventing contamination of the devices from outside organisms during bacterial growth is necessary.
Mass transport and the gas permeability of the selected materials must be considered when developing these devices. In the case of EEU bacteria, an anoxic environment needs to be maintained and choosing gas-impermeable materials is necessary. Conversely, streptomyces and actinomyces require the presence of oxygen. Thus, the availability of oxygen and in general of other gases and needed nutrients must be considered when designing the reactors.
When integrating various measurement systems, such as in-situ imaging, the optical characteristics of the chosen materials and the dimensions of the device become crucial design factors. Beyond the use of optically transparent materials, several considerations need to be addressed, such as ensuring that material thicknesses align with the focal length of microscope objectives. Moreover, when stacking different materials, understanding the refraction indices of the selected materials, and comprehending how different microstructures might influence the path of light is paramount in obtaining the desired imaging resolution.
The ultimate challenge remains in translating micro-level results to an industrial scale
While the above challenges can be addressed through engineering and design changes, the final challenge associated with miniaturized systems lies in the scaling up of results from the microbial level to the laboratory scale, and ultimately to an industrial level. The behaviors and interactions observed in highly controlled, microscale environments may not directly translate to larger, more complex systems due to differences in factors such as mass transport and population heterogeneity.
However, despite these difficulties, the insights gleaned from miniaturized systems can be invaluable. They offer a level of detail and control that is rarely achievable in larger setups, allowing us to uncover fundamental aspects of microbial behavior and biofilm formation. Even if direct scaling is not feasible, these insights can guide decision-making at the macro level. They can inform the design of more efficient bioreactors, help identify optimal operating conditions, and guide the development of better models for predicting microbial behavior at larger scales.

This work is licensed under Creative Commons Attribution-NonCommercial-NoDerivatives 4.0 International.