Ashley V. Makela and Christopher H. Contag from the Institute for Quantitative Health Science and Engineering, walk us through watching living therapeutics in action, including what imaging reveals about engineered endosymbionts
Advances in biomedical imaging have opened new windows through which we can watch dynamic biological processes in the context of intact organs and tissues of living subjects. As such, imaging has become integral to revealing fundamental insights and advancing applied scientific research.
Biomedical imaging is a field comprised of a variety of non-invasive imaging instruments and a myriad of molecular probes that can be deployed in animals and humans to detect, diagnose, and stage diseases, monitor therapeutic outcomes, and dissect molecular pathways in pathogenic processes.
Some imaging modalities can use intrinsic contrast in the body, such as magnetic resonance imaging (MRI), x-rays, and computed tomography (CT). In contrast, other modalities require imaging contrast agents, tracers, or reporter genes to visualize structures, cells, or processes, such as positron emission tomography (PET), optical imaging (fluorescent or bioluminescent), and magnetic particle imaging (MPI). Combinations of imaging modalities can enable visualizing changes in function in the context of structure, i.e., anatomy.
The molecular labels used in imaging can be deployed to observe the localization, survival, migration, and growth of cells in the body, and imaging can be used to guide the development of novel therapeutics. By watching the labels, we can visualize accumulation in a target tissue, monitor cargo being delivered to cells, and assess outcomes by tracking the therapeutic effect. Living therapeutics, such as immune cells and engineered bacteria, are particularly amenable to imaging because of their large capacity for carrying molecules that can be imaged and even engineered to produce labels that can be imaged.
Engineered endosymbionts as living therapeutics Engineered endosymbionts (EES) are bacteria that have been engineered to reside within the host cell cytoplasm through mechanisms allowing endosomal escape and survival in the cytoplasm. (1,2) The EES can be further engineered to control cellular function, through regulated and controlled expression of genetic circuits that interface with mammalian biology to direct cellular fates and function. Therapeutic EES are living, dynamic, large-capacity regulators that can be controlled, directed, and/or eliminated at will, and watching these processes with imaging tools enables their effective development as potent cellular control modules for directed therapy.
Bacteria have been used, and are being developed as cancer therapeutics, with the most common example being Bacillus Calmette-Guerin (BCG) to treat bladder cancer. (3) The mechanism of BCG in reducing progression and recurrence is still not entirely understood but is likely an effect of immune system enhancement. (4) EES would act similarly, but in addition, are engineered to act directly on the tumor-associated cells after they are internalized. In one example, EES have been engineered to control immune cells (5), such as those found in the tumor microenvironment. Other pathways that EES could be engineered to interfere with include cell division by encoding cell cycle regulators (e.g., p53) or by expressing tumor suppressors.
Delivery of EES to the cytoplasm is a challenge; however, since many immune cells have phagocytic properties, they naturally take up EES. This presented the opportunity to control immune function by EES (5) and was used to guide macrophages toward a pro-inflammatory M1 phenotype or an anti-inflammatory M2 phenotype. Inflammation plays a large role in the development, worsening, and progression of many diseases, and the use of immune-targeting EES could help control diseases where the immune system is dysregulated, such as arthritis, cancer, asthma, and type 1 diabetes.
Alternatively, EES can be used as a regenerative tool. EES could guide cellular reprogramming and be used to generate induced pluripotent stem cells (iPSC), followed by differentiation into various specialized cell types. One such possibility could be guided liver regeneration since EES accumulates initially in the liver after intravenous administration.
Using multi-step control, the EES could be used to convert the cells in which they are internalized (e.g., Kupffer cells of the liver) into iPSC, followed by activation of a different gene circuit to drive differentiation into hepatocytes. Using this approach, it may be possible to use EES to guide the regeneration of other cells in other tissues of the body. Guiding these complex processes with EES will be challenging, but developing these tools in the dark would be impossible. Imaging opens windows through which we can observe these living therapeutics as they interact with target cells and tissues in vivo.
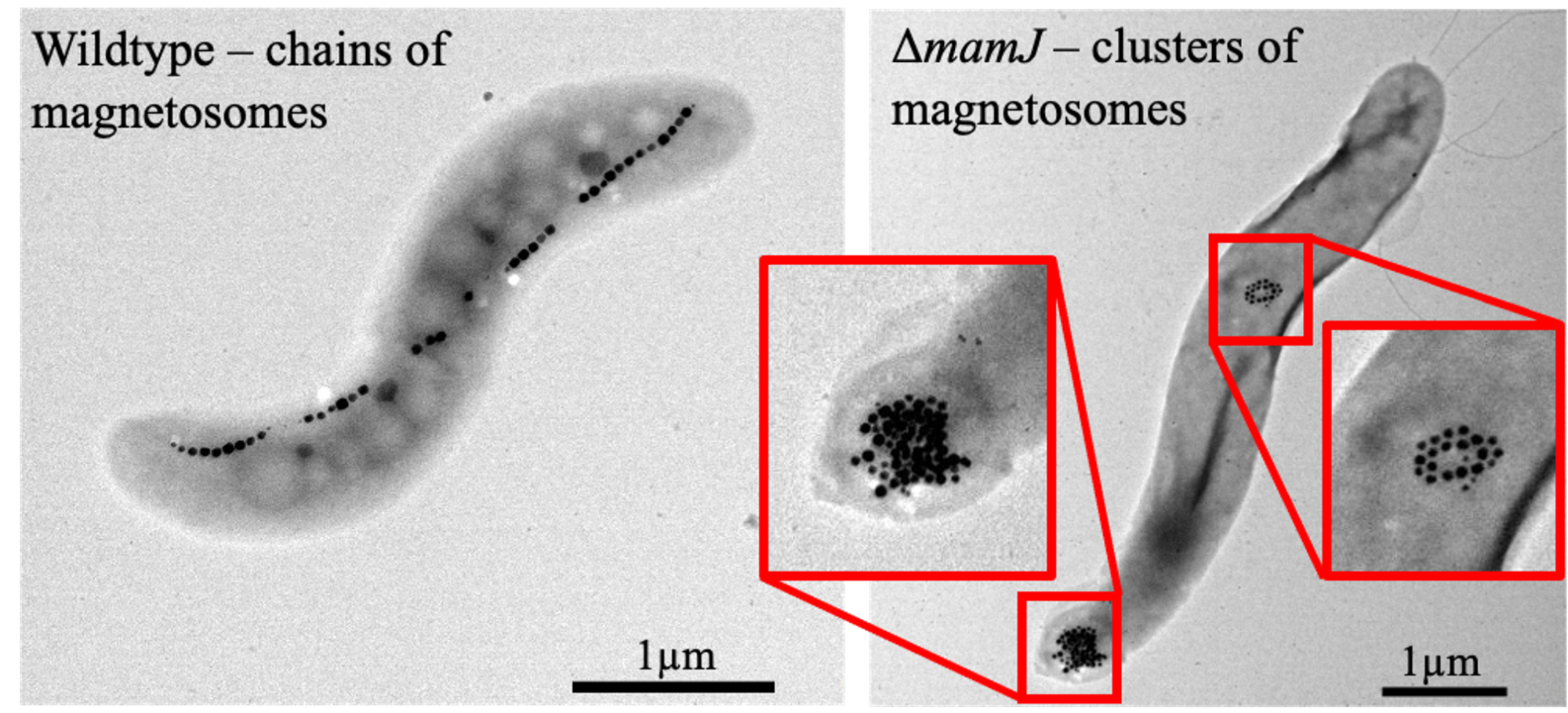
Imaging tools for endosymbionts
Using imaging technologies, EES can be observed in culture and within living subjects, and the strengths of each modality determine which to use based on intrinsic properties or the imaging label they contain. EES can be stained using fluorescent dyes, engineered to express their own fluorescent or bioluminescent proteins, and some bacteria create their own contrast, i.e., magnetic particles in magnetotactic bacteria (MTB) (Figure). Fluorescently labeled bacteria can be used to understand EES-cell interactions in culture using fluorescent microscopy or imaged in vivo using optical signals. EES can be engineered to create their own fluorescent proteins so that they can be imaged over time without the concern of losing the dye. However, the visualization of fluorescent bacteria can be limited by the muscle, skin, and fur absorbing and scattering of the optical signals when used in vivo.
Bioluminescent imaging (BLI) uses proteins that emit visible light (i.e., luciferases), and EES engineered to express these proteins glow in the dark. Bacteria can be engineered to emit light through bacterial luciferase, called Lux, which can provide signals to locate the EES and, further, can serve as an orthogonal measure of viability. The bacterial luciferase is a sustained reaction in the EES, and the light only disappears when the bacteria are dead. Both fluorescent proteins and luciferases can be engineered into EES with genetic light switches so that these proteins serve as indicator lights, revealing cellular function.
MTB naturally synthesize iron nanoparticles called magnetosomes, and using these as the foundation of the EES presents an opportunity to combine imaging with a therapeutic. The magnetosomes are superparamagnetic, meaning they can be imaged using MRI or MPI. With MRI, they will appear as dark regions on the image, which are called signal voids. (6) Conversely, with MPI, they will appear as a positive contrast. These MTBs can be further manipulated to change the shape of their magnetosome structure, improving MPI (7) and enabling magnetothermal control of function.
Image-guided development of EES applications
There are challenges when using EES to target specific organs or sites within the body. However, bacteria naturally accumulate in tumor sites, eliminating the need for further manipulation of the EES when acting as a cancer therapeutic. Further, systemic administration of the EES allows for the accumulation in phagocytic cells of the tumor, liver, and spleen – these are the current target cells and tissues for EES-based therapies.
Imaging reveals where EES accumulates after administration into a living subject. EES, which have optical reporters, or MTB (Figure), can be imaged over relevant periods of time to better understand their biodistribution, longevity, kinetics, and efficacy.
Magnetoendosymbionts, i.e., EES based on magnetotactic bacteria, have magnetosomes that provide the contrast needed to determine localization and accumulation of EES (Figure). Following this, a non-invasive alternating magnetic field can be applied to induce magnetothermal energy, either activating gene expression (8) or, if needed, eliminating the EES using heat.
EES, which contain an optical reporter, will provide real-time monitoring of the accumulation of the EES, revealing information about how long the EES remain viable or how quickly they are cleared from normal healthy tissue. For example, when bacteria are administered systemically into a tumor-bearing mouse, they are eliminated by healthy tissue, whereas they remain viable within the tumor. (9)
Further, the development of tools to identify EES-expression of gene circuits allows for real-time feedback. In a genetically modified system, the EES expression of the gene circuits would also trigger light production, verifying the production and delivery of the gene. Engineering living systems for the benefit of mankind is greatly enhanced by using imaging to guide their development and assess the outcome of these living therapeutics.
References
- Kulkarni, S. H.; Contag, C. H. Engineered endosymbionts as novel cancer therapeutics. Open Access Government, April 2024; pp. 126-127.
- Contag, C. H. Engineered endosymbionts for cellular control. Open Access Government, January 2024; pp 52-54.
- Morales, A.; Eidinger, D.; Bruce, A. W. Intracavitary Bacillus Calmette-Guerin in the treatment of superficial bladder tumors. The Journal of urology 1976, 116 (2), 180-182.
- Han, J.; Gu, X.; Li, Y.; Wu, Q. Mechanisms of BCG in the treatment of bladder cancer-current understanding and the prospect. Biomedicine & Pharmacotherapy 2020, 129, 110393. DOI: https://doi.org/10.1016/j. biopha.2020.110393
- Madsen, C. S.; Makela, A. V.; Greeson, E. M.; Hardy, J. W.; Contag, C. H. Engineered endosymbionts that alter mammalian cell surface marker, cytokine and chemokine expression. Communications Biology 2022, 5 (1), 888.
- Makela, A. V.; Gaudet, J. M.; Schott, M. A.; Sehl, O. C.; Contag, C. H.; Foster, P. J. Magnetic particle imaging of macrophages associated with cancer: Filling the voids left by iron-based magnetic resonance imaging. Molecular imaging and biology 2020, 22, 958-968.
- Makela, A. V.; Schott, M. A.; Madsen, C. S.; Greeson, E. M.; Contag, C. H. Magnetic Particle Imaging of Magnetotactic Bacteria as Living Contrast Agents Is Improved by Altering Magnetosome Arrangement. Nano Lett 2022, 22 (12), 4630-4639. DOI: 10.1021/acs.nanolett.1c05042.
- Greeson, E. M.; Madsen, C. S.; Makela, A. V.; Contag, C. H. Magnetothermal Control of Temperature-Sensitive Repressors in Superparamagnetic Iron Nanoparticle-Coated. ACS Nano 2022, 16 (10), 16699-16712. DOI: 10.1021/acsnano.2c06239.
- Min, J.-J.; Nguyen, V. H.; Kim, H.-J.; Hong, Y.; Choy, H. E. Quantitative bioluminescence imaging of tumor-targeting bacteria in living animals. Nature Protocols 2008, 3 (4), 629-636. DOI: 10.1038/nprot.2008.32.

This work is licensed under Creative Commons Attribution-NonCommercial-NoDerivatives 4.0 International.