Dr F. Barry Dunning, Sam and Helen Worden Professor of Physics at Rice University in Houston, explains some key discoveries about the structure of atoms
Until the middle of the nineteenth century, little was known of the structure of atoms. The first step in unraveling this mystery was provided by the pioneering Swedish spectroscopist J. A. Angström who studied the lines in the spectrum of hydrogen. A formula that described these lines was developed by J. A. Balmer, but it was another Swedish spectroscopist J. R. Rydberg who developed a more general formula that could describe the spectra emitted by a variety of atoms.
Rydberg’s formula was central to the development of the semi-classical Bohr model of the hydrogen atom early in the twentieth century which pictured the atom as a negatively- charged electron moving in a classical circular orbit about a positively-charged nucleus (the proton), the two being bound together by electro- static forces.
This model was successful in explaining the hydrogen spectrum and showed that the
electron could occupy one of a series of allowed excited states whose properties are defined by
the so-called principal quantum number, n. In particular, the radius of the electron orbit increases rapidly with n, scaling as n2. Atoms in their ground state have diameters of less than one nanometer. However, highly-excited states with values of n greater than 1,200 have been seen both in nature and in the laboratory and possess diameters of several hundred micrometers, comparable to the width of a human hair.
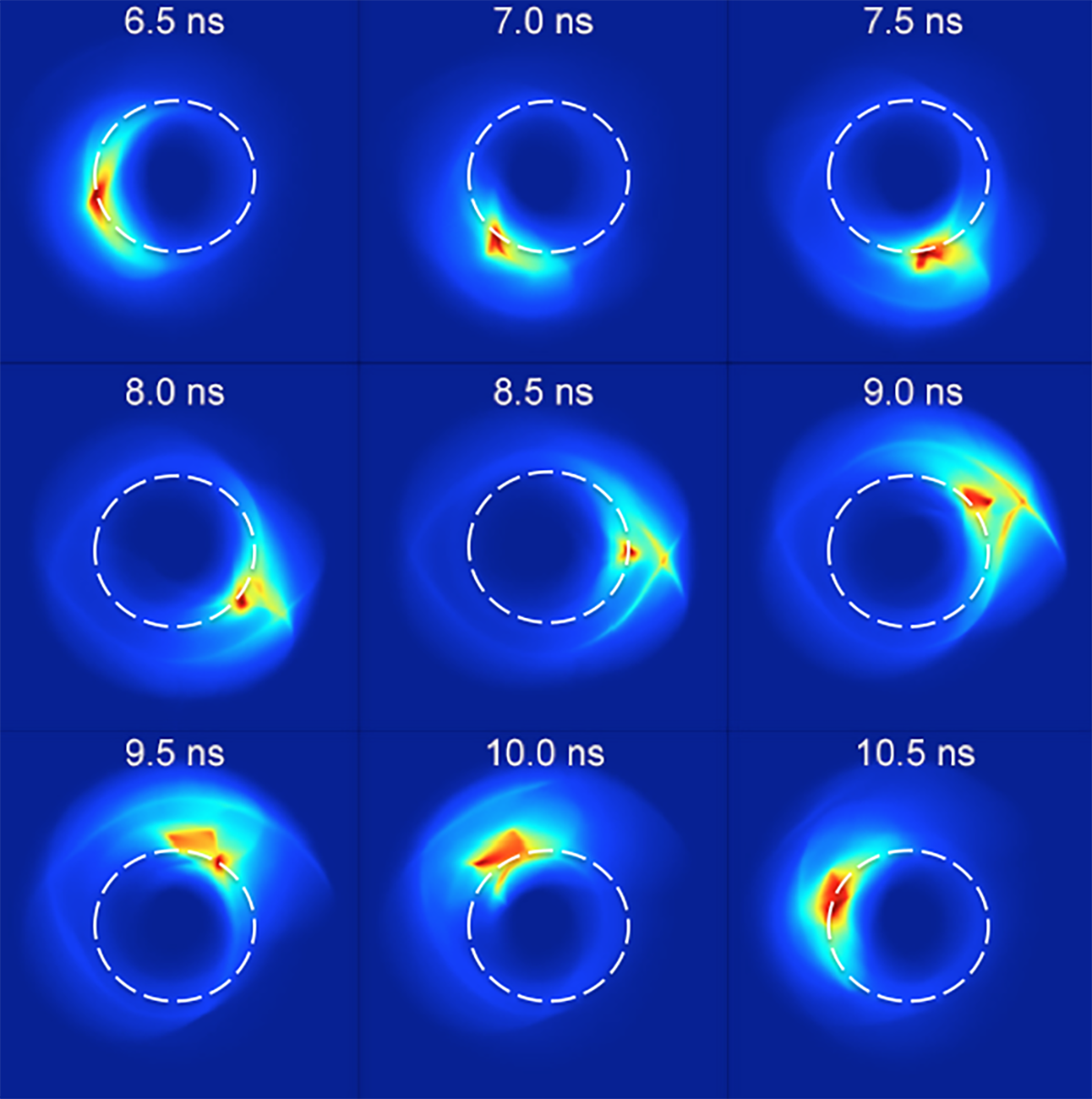
Such atoms thus represent true giants on the atomic landscape. However, since the bulk of
the atomic volume is occupied by only a single electron, high-n atoms, termed Rydberg atoms, are transparent and not visible to the naked eye. Although the Bohr model has been supplanted by more modern quantum theories, it still provides valuable insights into the behavior of the Rydberg states of even complex multi-electron atoms because, in essence, they too comprise an excited electron in distant orbit around the nucleus and remaining inner electrons which together form a compact positively-charged “core ion.” Given their large size, Rydberg atoms form a valuable bridge between the microscopic world described by quantum mechanics and the macroscopic world ruled by Newton’s laws.
Many other properties of Rydberg atoms depend strongly on n. The electron orbital period increases as n3 and can, for high-n atoms, approach several tens of nanoseconds. As n increases, the energy required to strip the excited electron from the atom , termed its binding energy, decreases rapidly, varying as 1/n2, and the atoms become increasing fragile. Furthermore, as n becomes large and the electron is able to travel farther from the nucleus, its motion becomes increasingly sensitive to external perturbations in the form of electric (or magnetic) fields, or collisions. Nevertheless, Rydberg lifetimes are, by atomic standards, quite long. Whilst the natural lifetimes of low-lying excited states are typically a few nanoseconds, those for high-n states can amount to a millisecond or longer
Highly-excited Rydberg atoms have exaggerated properties quite unlike those normally attributed to atoms in ground or low-lying excited states. As is now discussed, these novel properties fuel much of the current interest in such species and underpin their broad-ranging applications.
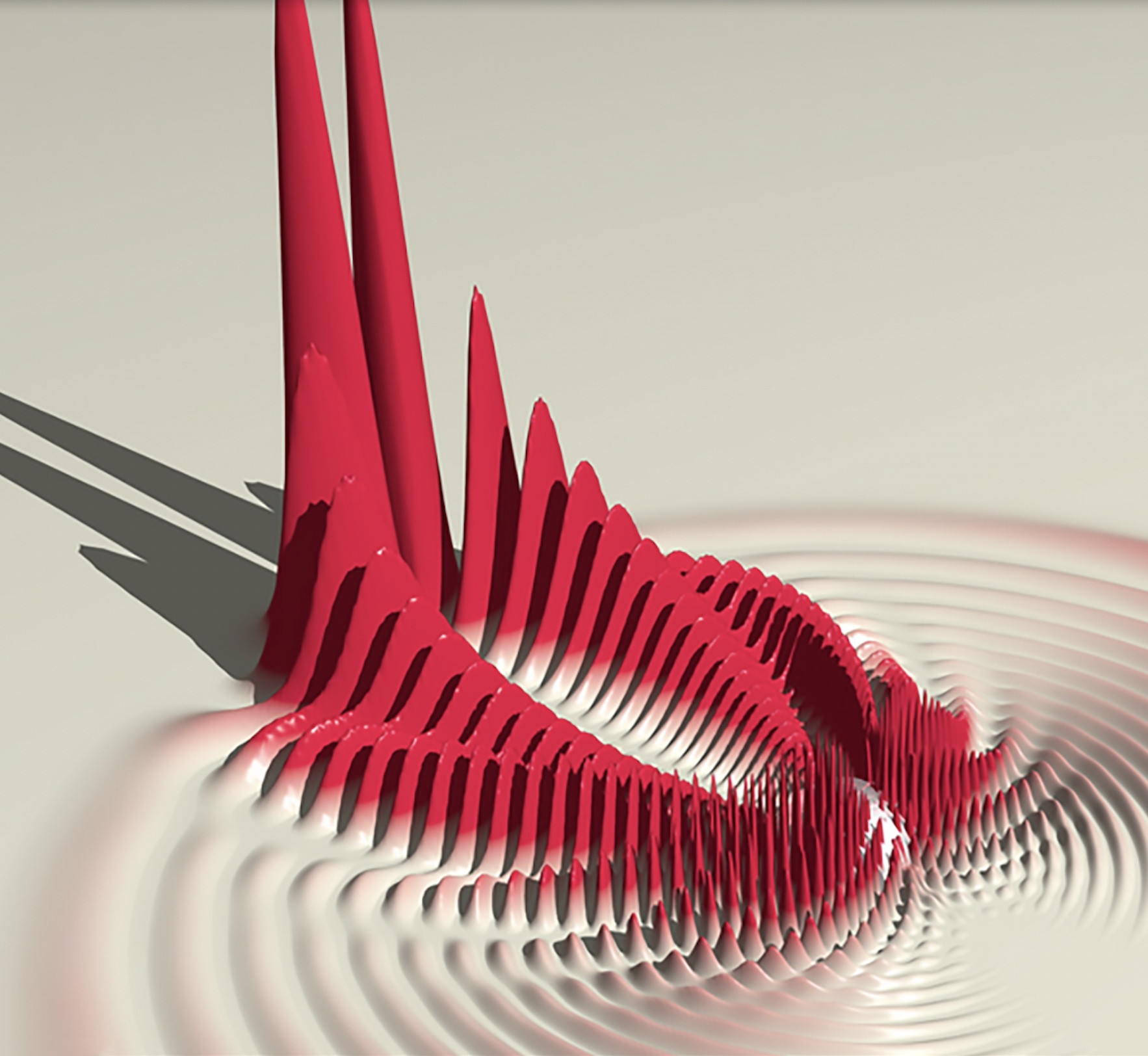
Chemical properties
Given their unusual physical characteristics it is not unexpected that Rydberg atoms also possess unusual chemical properties. In collisions with other neutral atoms or molecules such atoms frequently behave not as an atom but rather as a pair of well-separated particles, the excited electron and the core ion, each of which interacts independently with a target particle. The energy of the “essentially- free” electron, equal to its binding energy, is low and Rydberg collisions provide a valuable platform to study electron- molecule collisions at effective collision temperatures extending below 1K – much lower than those accessible using any alternate technique. This has enabled detailed study of electron capture by a variety of fluorinated molecules. Such capture results in their use, for example, to prevent breakdown in electrical equipment and to extinguish fires. Electron capture, however, also frequently leads to molecular breakup with the formation of negative ions and highly- reactive fragments whose subsequent interactions are responsible for their environmental damage and their toxicity. Rydberg collisions have provided valuable new insights into the dynamics of electron capture by such species and the resulting fragmentation pathways.
Remarkably, under appropriate conditions, the negative ion that results from electron capture can remain electrostatically-bound to the Rydberg core ion. The ion pair orbit at a large separation, creating a giant neutral molecule, termed a heavy-Rydberg molecule. Such molecules possess many of the extreme characteristics of Rydberg atoms except that the electron is replaced by a much-heavier negative ion.
Atomic engineering
Pulsed electric fields whose duration is much less than the Rydberg electron orbital period are now readily generated providing access to detailed study of atomic behavior in the so-called ultra-fast, ultra-intense regime more typical of ground-state atoms subject to high-power femto- and atto-second laser pulses, the use of exotic Rydberg atoms replacing the use of exotic laser systems. Research has demonstrated that Rydberg atoms subject to a periodic series of short impulsive electrical forces, or “kicks,” provide an ideal system with which to explore non-linear dynamics and classical and quantum chaos, as well as to highlight the underlying geometrical structure of phase space. “Designer atoms” have been engineered through use of carefully-tailored pulse sequences. Such an approach has been used, for example, to localize the Rydberg electron in a near-circular orbit and create a “Bohr-like” atom as well as to model features of the solar system within an atom. Indeed, the level of control that can be exercised suggests it will be possible in the future to create “planetary atoms” in which two localized highly- excited electrons orbit the core ion, thereby mimicking a tiny solar system. Other future possibilities include the imprinting of information in a Rydberg atom allowing it to serve as a memory element.
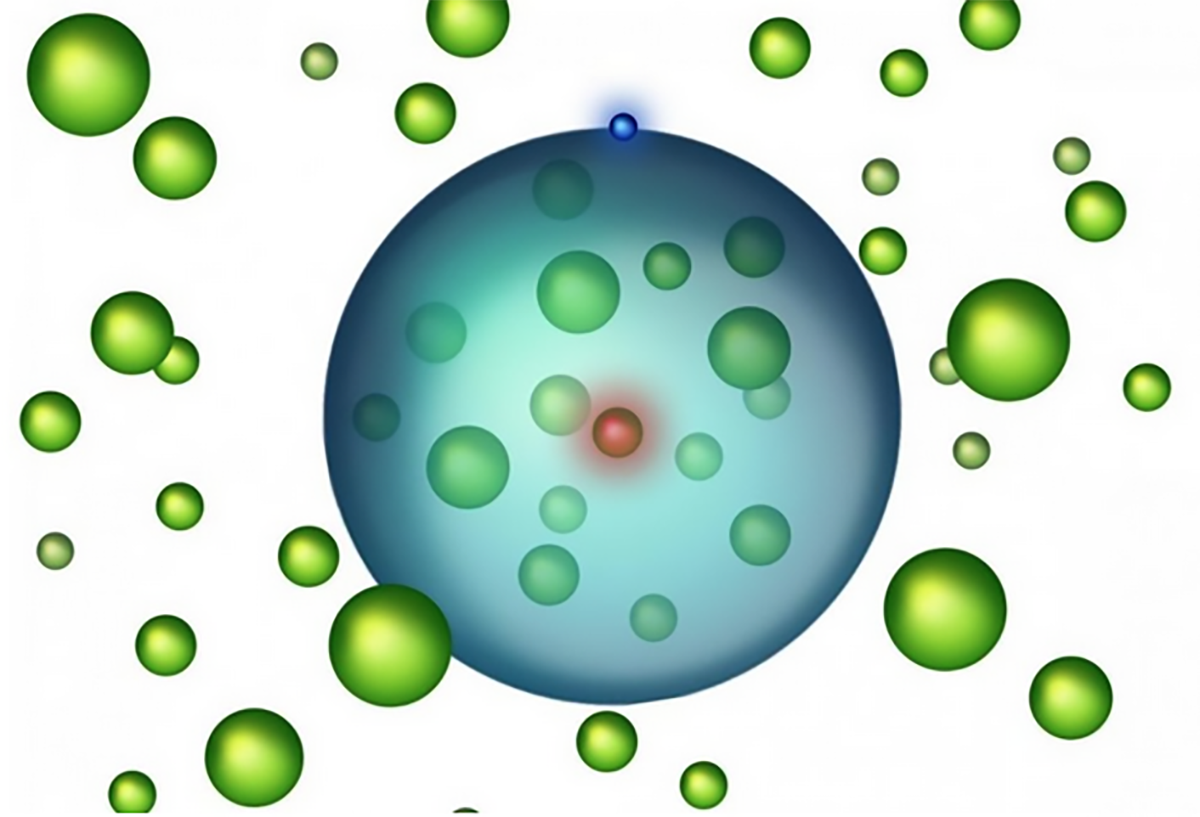
Atoms within an atom
Rydberg atoms allow formation of a whole new class of ultralong-range molecules comprising one, or more, ground-state atoms bound within the electron orbit. Atomic binding results from scattering of the Rydberg electron from the bound atom(s) but is very weak. In consequence such molecules are not stable at room temperature.
However, advances in laser cooling and trapping now allow creation of cold dense gases with temperatures of one microkelvin or less in which to create and study such species. Initial experiments focused on the creation of dimer molecules comprising one Rydberg atom and one bound ground-state atom. The likelihood of molecule formation depends on having atom pairs with initial separations comparable to the size of the parent Rydberg atom. Dimer formation therefore provides a valuable tool with which to probe spatial separations in quantum gases and over a range of interparticle spacings not accessible using any alternate approach. Such studies have revealed effects of quantum statistics such as particle “bunching” in a gas of bosons and “anti-bunching” in a gas of fermions.
In Bose-Einstein condensates the atom densities are so high that molecules can be created in which the electron corrals tens, or even hundreds, of ground-state atoms within its orbit. The bound atoms represent a novel form of “quantum matter” that provides a new avenue to explore how collective atomic interactions lead to emergent phenomena such as the creation of “quasiparticles” similar to those that govern the electric, magnetic, and optical properties of many materials. Rydberg molecules are furnishing a powerful window into the world of many-body interactions in solid-state systems and in quantum materials in general.
The use of Rydberg atoms as sensors
Rydberg atoms make particularly sensitive electric field sensors because they are strongly perturbed by even very weak external fields. Furthermore, since all atoms of a particular species are identical, Rydberg-based electric field sensors are readily replicated secure in the knowledge that each will respond identically and share a common, time-in- dependent calibration that can be referenced directly to internationally-defined standards.
Static electric fields are measured through the changes they induce in the Rydberg energy level structure. These changes can be detected using laser or microwave spectroscopy and permit detection of fields as low as a few microvolts
per centimeter. Oscillating electric fields can be observed through the transitions they induce between different Rydberg levels. Such transitions are particularly strong and can be readily identified by measuring the distribution of product Rydberg states.
Another approach is to harness an effect termed electromagnetically-induced transparency which involves a cloud of atoms through which is directed a “probe” laser beam tuned to a frequency the atoms would normally strongly absorb together with a superposed “control” beam tuned to excite atoms to a Rydberg state. With careful choice of the control beam frequency its presence can lead to transmission of the probe beam through the otherwise opaque atom cloud. The presence of even very small external fields can lead to marked changes in transmission which facilitates the detection of electromagnetic waves over a wide portion of the electromagnetic spectrum that extends from radio frequencies to the near infrared with applications in, for example, communications and radar. Rydberg atoms sensors are remarkably sensitive and have been shown, through so-called quantum non-demolition measurements, to allow the detection of fields at the ultimate single- photon level without even absorbing the photon.
Quantum technologies
Interactions between Rydberg atoms, which are millions of times stronger than those between ground state atoms, underlie the development of Rydberg-based quantum gates, or qbits, for use in a possible quantum computer. This work exploits “dipole blockade” in which resonant excitation of one atom to a Rydberg state shifts the energies of neighboring atoms within some “blockade radius” inhibiting their subsequent excitation. Ground- state atom pairs are initially positioned within one blockade radius of each other using tightly- focused laser beams as very precise “optical tweezers.” Gate action then relies on conditional logic where excitation of the second atom is governed by excitation of the first. Rydberg-based gates have proven fast and reliable with considerable promise for use in quantum logic devices.
Rydberg-Rydberg interactions are also central to many efforts in quantum simulation, i.e., simulating the behavior of complex quantum systems or materials, behavior which is frequently too complex to model even on a modern computer.
To accomplish this, optical tweezers, as well as optical lattices formed by intersecting laser beams, are used to assemble one-, two-, and even three-dimensional ordered arrays of Rydberg atoms with precise control of their geometry and spacing. Such arrays can be engineered to match the arrangement of atoms within some solid and thus probe the behavior of the solid they mimic, especially properties such as superconductivity or magnetism that result from collective internal atom-atom interactions. The effects of defects introduced by removing atoms from the array or substituting “impurity” atoms can be explored. Interestingly, artificial structures not found in nature can also be constructed and their properties examined.
Recently, microwave-driven transitions between multiple Rydberg levels within a single atom have been harnessed to mimic the behavior of particles held in a real-space lattice thereby creating a novel “synthetic lattice.” Such “synthetic dimensions” furnish new opportunities for simulation of quantum matter and can be used in conjunction with real space lattices to explore higher dimensional systems not accessible using either approach separately. Rydberg atom studies are providing new insights into the behavior of condensed matter systems and promise the design of new materials with enhanced electronic, magnetic, optical, and even thermal properties.
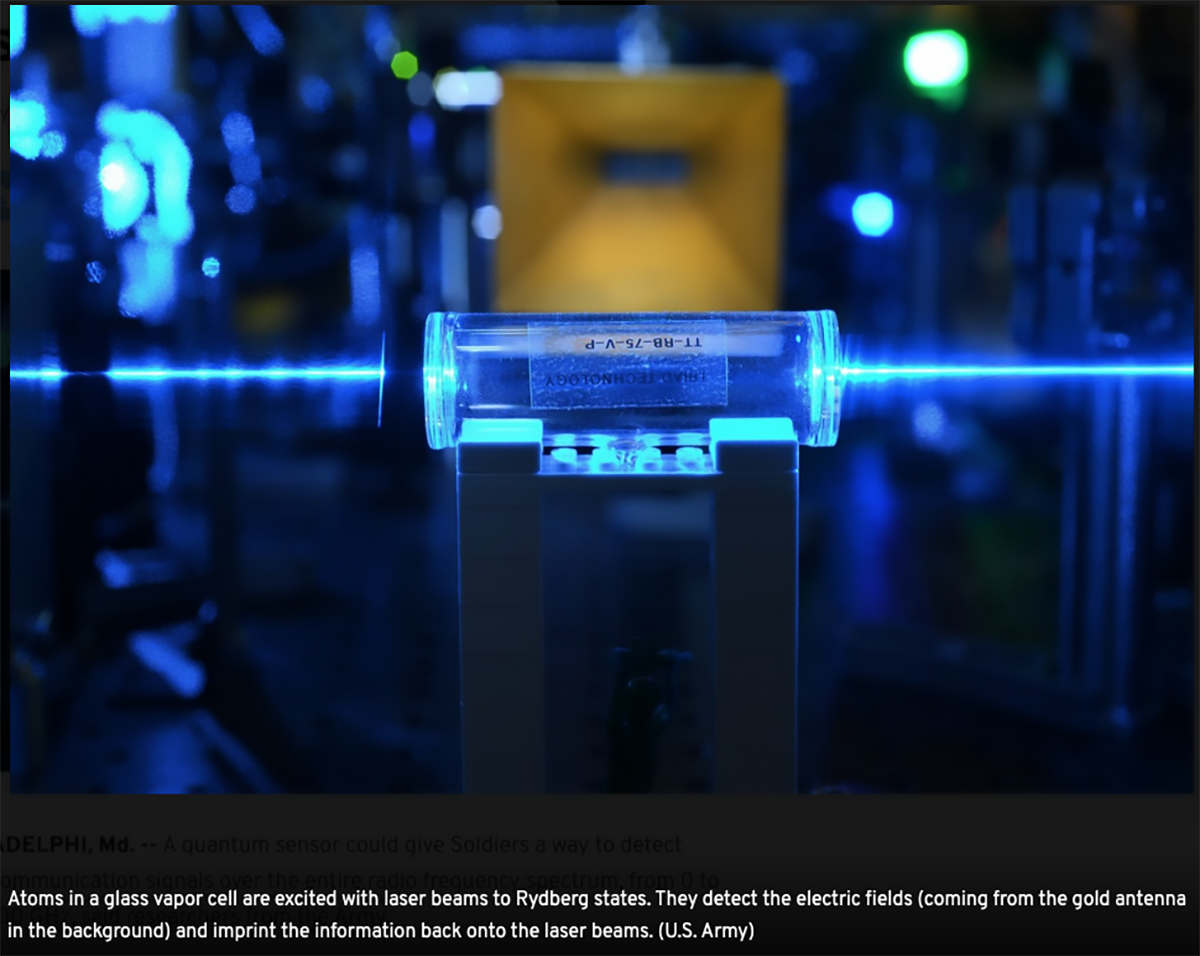
The future of Rydberg atom research
Remarkably, despite their relatively large size, Rydberg atoms provide a valuable stage upon which to explore the quantum world and complex quantum behaviors. Advances in experimental technique continue to yield exciting new discoveries which will lead to ever-widening applications in critical areas of technology such as sensing, quantum simulation, quantum information, and materials design.
Dr. F. Barry Dunning is the Sam and Helen Worden Professor of Physics at Rice University in Houston, Texas.