Plastics have greatly benefited humanity, enabling many significant advances in medicine, electronics, aerospace, construction, food packaging, and sports. However, there is growing concern that plastics may also be responsible for significant harm to the earth’s environment and human health
This alarm has emerged because plastics, especially micro- and nanoplastics (MNPs), have been found almost everywhere scientists have investigated, including the polar ice caps, the depths of the Mariana Trench in the western Pacific, the air we breathe, the food and water we consume, and every tissue in our bodies. This concern has intensified due to emerging data indicating that higher levels of MNPs in human tissues are correlated with increased risk of disease, alongside findings from experimental models indicating that MNPs can trigger significant inflammation and oxidative stress, both of which are implicated in the development of many adverse health effects.
Detection is only the first step; determining whether MNPs are harmful remains a key question. What is known is that the effects of MNPs vary based on their chemical and physical properties. Chemically, plastics are complex, highly heterogeneous synthetic materials. Over 98% of plastics are made from fossil carbon (e.g., coal, oil, and gas), which is used to synthesize carbon-based chains (polymers) composed of smaller repeating units (monomers). Chemical additives are often integrated into plastic polymers to provide specific properties such as color, flexibility, stability, water repellence, flame retardation, and ultraviolet resistance. As a result, there is a range of different plastics with distinct properties, including polyethylene terephthalate (PET), polyvinyl chloride (PVC), and polystyrene (Styrofoam). Many of the chemicals added to plastics, including phthalates, bisphenols, per- and poly-fluoroalkyl substances (PFAS), brominated flame retardants, and organophosphate flame retardants, are known toxicants that promote cancer, organ-specific toxicities, and endocrine disruption.
The size of plastics is also important in determining their potential to cause harm. Macroplastics are defined as plastics larger than 5 millimeters; microplastics (MPs), between 5 millimeters and 1 micrometer; and nanoplastics (NPs), less than 1 micrometer. Due to their small size, NPs are of particular concern. They are easily transported longer distances in the environment, are able to infiltrate deep into tissue and penetrate cellular barriers, and their larger surface area allows for greater adsorption of toxic chemicals.
Plastic production has increased almost exponentially since World War II.
Globally, about 400 million tons of plastics are produced each year, with production projected to more than double by 2050. Even if all plastics production were suddenly stopped today, the estimated 5 billion tons of existing plastics in landfills and the environment would continue degrading into MNPs indefinitely. This sobering fact underscores the current surge in research to develop rigorous methods for analyzing MNPs in environmental samples, including human tissues, and to understand their interactions with biological targets. Here, we provide a snapshot overview of this evolving landscape with a focus on NPs.
Environmental NPs
NPs can be categorized as primary or secondary. Primary NPs are those manufactured specifically at the nanoscale for industrial or consumer uses. One example is nanoscale plastic particles used in paints and coatings. These particles are intentionally engineered for automotive paints, protective industrial coatings, and even some household paints, to provide a smooth finish and improve UV resistance. Another example is the encapsulation of fertilizers, drugs, and cosmetics in NPs to slow their release in the environment or human body. This slow release delivery increases the half-life of the chemical, which increases the time between repeat administration. NPs are released from these and other NP products into the environment during manufacturing, application, or wear.
Secondary NPs are formed by the breakdown of larger plastics, often as a result of physical, chemical, or biological processes. Common sources include discarded bottles, food packaging, tires, and synthetic textiles (like polyester, nylon, and acrylic based cloth), which are degraded by sunlight, heat, and mechanical wear. NPs are highly persistent and mobile. Their chemical stability allows them to remain intact for extended periods, while their small size enables them to move easily through soil, freshwater systems, marine environments, and even the air. Thus, NPs can travel long distances, infiltrating ecosystems far from their original source. Secondary NPs do not readily degrade and can persist in the environment for decades, posing long-term challenges for ecosystems and human health.
Humans are exposed to NPs through both dietary and non-dietary pathways. NPs accumulate in seafood, fruits, and vegetables due to plastic debris in soil and water, thus, consumption of contaminated food is a significant source of human exposure. Food packaging and processing also contribute to dietary exposures, with single-use plastics, containers, and wraps leaching NPs into food during handling, storage, and heating. Non-dietary pathways of exposure include inhalation of airborne NPs, which are released into the air from tire wear, industrial emissions, and synthetic textile fibers. These particles can penetrate the respiratory system and potentially enter the bloodstream. Cosmetic
and personal care products are another source, as nanoscale plastic additives in deodorants, shampoos, and exfoliating microbeads can enter wastewater systems or be absorbed through the skin. Everyday consumer goods, such as disposable razors and synthetic clothing, also release NPs through wear and fragmentation.
Detection of nanoplastics (NPs) in human tissues
While detection methods are improving, identifying and quantifying NPs remains technically challenging due to their tiny size and complex interactions in biological and environmental samples. Current methods like electron microscopy, Raman spectroscopy, and mass spectrometry can readily detect larger MPs but often lack the sensitivity and specificity needed for NPs. Nonetheless, using these techniques, MPs have been detected in nearly all human populations and in every part of the human body studied to date, including organs (lungs, liver, spleen, kidneys, heart, and brain), bodily fluids (blood, semen, breast milk, and mucus), and excretions (stool and urine).
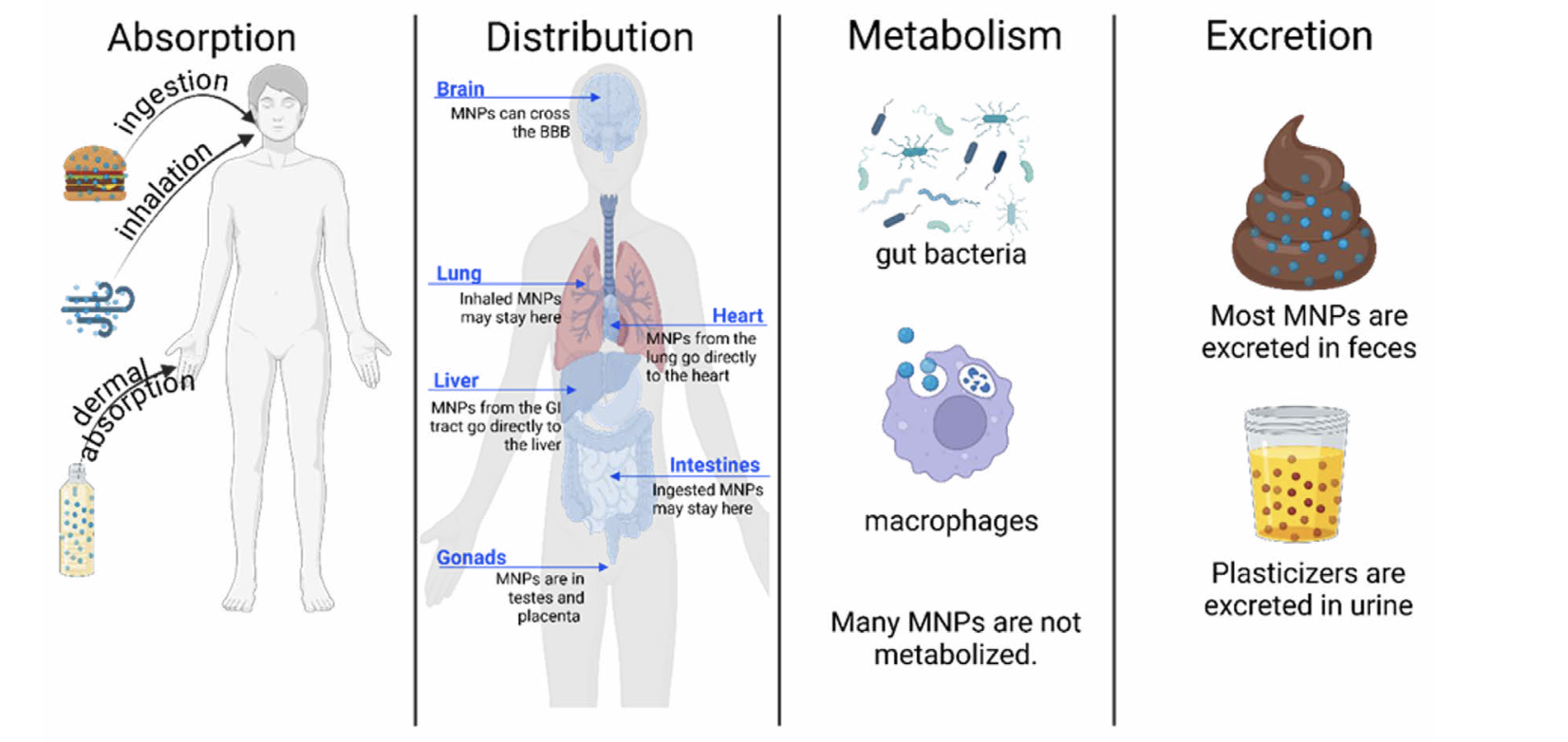
The widespread detection of MPs in humans suggests that it is highly probable that NPs are also present in these same tissues – and potentially in greater numbers due to their enhanced mobility and ability to penetrate biological barriers. Recent human studies are beginning to confirm this. Furthermore, experimental animal studies have shown that following dietary ingestion, NPs reach sensitive organs, including the liver, kidneys, brain, and reproductive tissues. NPs may also be able to cross the intact blood-brain barrier.
Of utmost importance in understanding NP toxicity is understanding the absorption, distribution, metabolism, and excretion (ADME) of NPs (Figure 1). As indicated earlier, the size and chemical composition of plastics plays a crucial role in absorption. Relative to MPs, NPs can more readily cross cellular barriers in the skin, gastrointestinal (GI) tract, and lungs to gain access to the blood system. Once in the blood, the hydrophobic (“water-hating”) NPs often bind with hydrophilic (“water-loving”) proteins to form a shell referred to as a “protein corona”, which facilitates their distribution throughout the body and increases their ability to cross cell membranes. It remains controversial as to whether NPs can be metabolized by host enzymes, gut microbiota, or immune cells, but it is currently understood that most NPs are eliminated in feces, particularly those that are ingested via the diet, while the chemical additives that coat the NPs are more likely to be excreted in urine.
However, because NPs remain difficult to isolate, characterize, and quantify reliably, the data currently available regarding NPs in human tissues remains uncertain. Investments in research to improve NP detection methods are crucial to understanding their distribution, accumulation, and potential health consequences in humans.
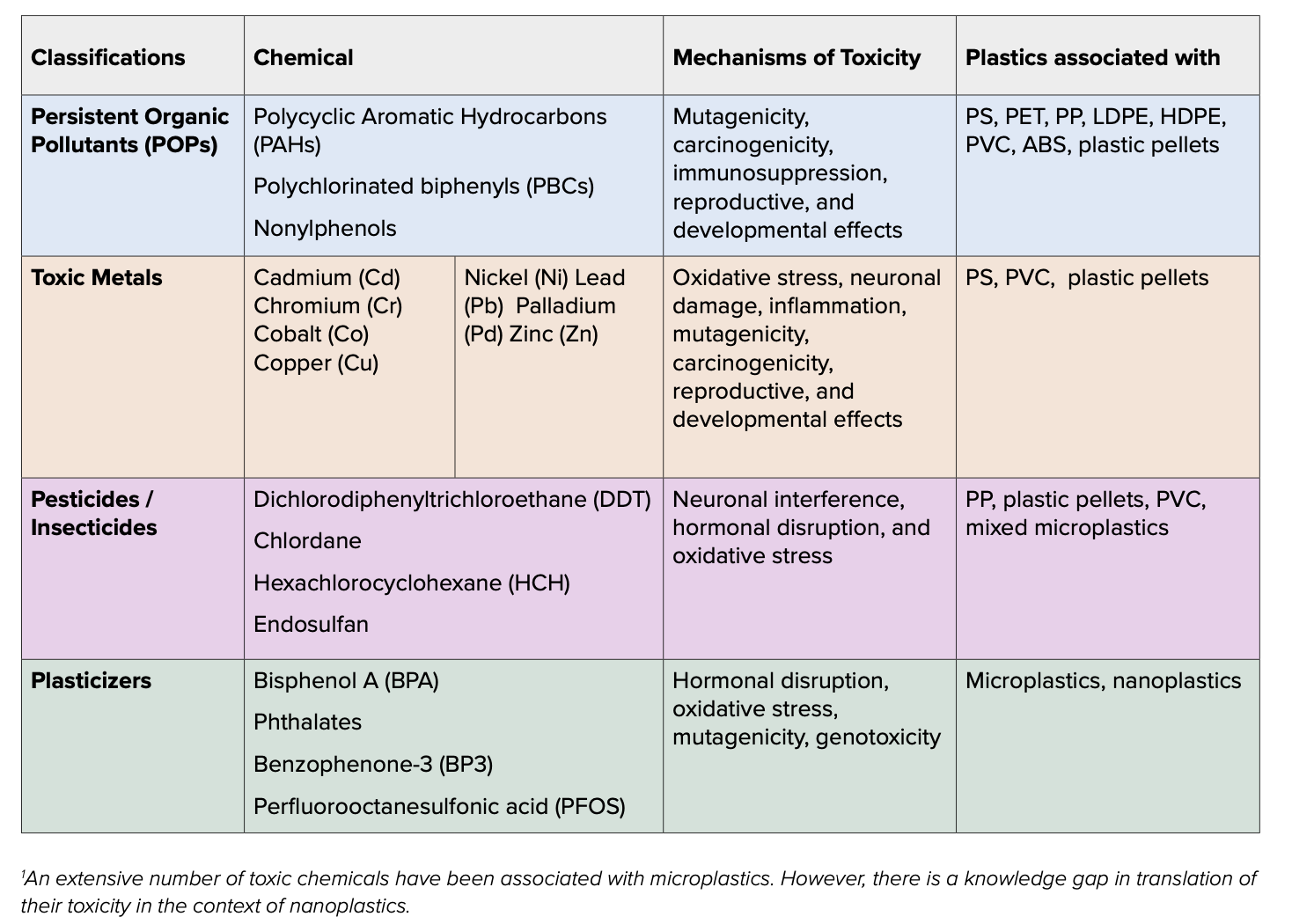
Potential mechanisms by which NPs may cause toxicity
NPs may induce toxicity in the body via multiple mechanisms, which can be categorized into three mechanistic categories: physical, chemical, and biological. The plastics’ physical properties can lead to inflammation, cell/tissue damage, and obstruction of cellular processes. NPs are also known to associate with chemical pollutants and biological toxins, acting as “Trojan horses,” carrying these toxic compounds throughout the body.
In the environment, MNPs are exposed to a chemical “soup” of pollutants, including persistent organic pollutants (POPs), toxic metals, and pesticides. Polycyclic aromatic hydrocarbons (PAHs), which are carcinogenic compounds produced in vehicle exhaust and cigarette smoke, are strongly associated with polystyrene (PS) and poly(ethylene terephthalate) (PET) NPs, and facilitate their transport into cells. MNPs are also known to associate with numerous POPs, metals, PFAS, and more, and the combination of the MNPs with the chemicals have been shown to have greater harmful effects on organisms than the toxicants themselves.
NPs also have a relatively large surface area and highly reactive surface, which allows them to interact with a large number of substances, including metals, organic and inorganic compounds, and other NPs (Table 1). These interactions can also cause NPs to form a larger particle (aggregate) with a wide variety of chemicals, some of which may be toxic. The unique compositions of these aggregates may influence their toxicities, making it difficult to define the general toxic effects across different environmental NPs. Some NPs on their own are small enough to be transported or taken up into cells, potentially bringing in toxic chemicals as well. The reactive surfaces of NPs can cause interactions with cell membranes, leading to the destruction of the membrane and subsequent cell death. Cell damage and death stimulate an inflammatory response, which, if persistent, can promote various diseases. Inside the cell, NPs may cause damage to the cell through either direct action or by releasing reactive, toxic chemicals attached to their surface. NPs can promote oxidative stress by interfering with mitochondrial function and/or depleting cellular antioxidants, resulting in harmful levels of reactive oxygen species (ROS). They may also disrupt the function of the endoplasmic reticulum (ER) resulting in aberrant protein misfolding, which can lead to ER stress and potentially cell death.
Another area of concern in NP toxicity is the adsorption and transport of microbes and viruses on NPs, as has been seen with larger microplastics. Bacteria are capable of adhering to the surface of MPs to form biofilms, which create a sticky, hydrophobic surface for the bacteria to colonize the microplastic. This biofilm allows for the potential transport of harmful bacteria into the body when ingested or inhaled, as well as the co-transport of highly toxic pollutants, like per- and polyfluoroalkyl substances (PFAS), embedded in the biofilm. SARS-CoV-2 and influenza A viruses have been shown to adhere to MPs, which, if inhaled, can spread infections by these viruses. However, it has not yet been determined whether bacteria or viruses can adhere to the surface of NPs or agglomerate with NPs. Bacteria may be too large (0.1 – 10 μm) to attach to individual NP surfaces, but agglomerates could provide enough surface for some bacteria to colonize. The average size of viruses ranges between 20 – 400 nm, which suggests that viruses may be small enough to attach to NPs.
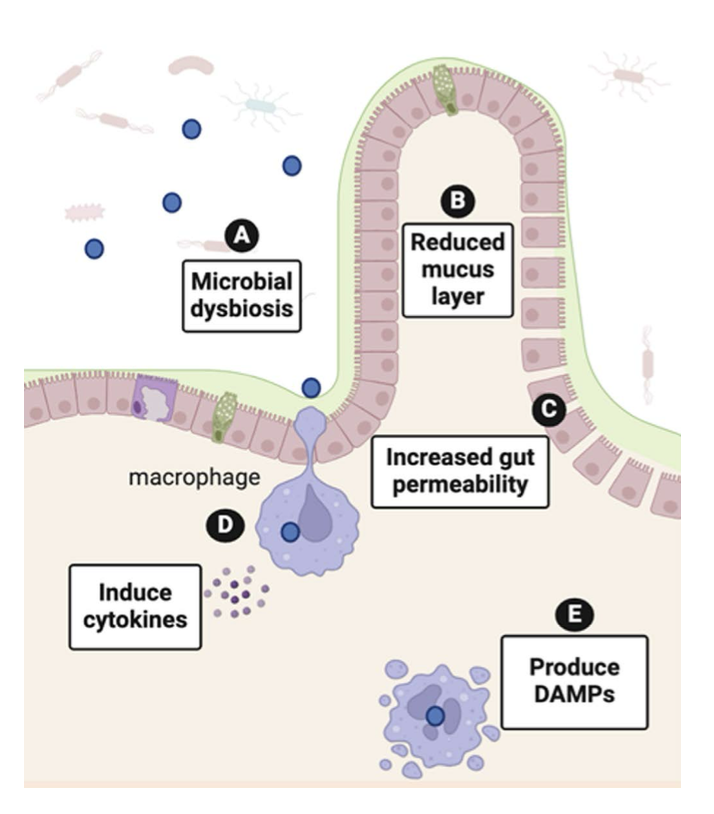
What is the evidence that NPs cause adverse human health effects?
Increasingly, NPs are being linked to adverse health effects in humans. Recent epidemiologic studies of occupationally exposed populations found that plastic production workers had an increased risk of diverse cancers, neurotoxicity, and decreased fertility, while plastic recycling workers experienced increased rates of cardiovascular disease, toxic metal poisoning, neuropathy, and lung cancer. Communities that live next to plastic production and waste disposal sites exhibit an increased risk of premature birth, low birth weight, asthma, childhood leukemia, cardiovascular disease, chronic obstructive pulmonary disease (COPD), and lung cancer. The emerging data suggests that life stage is a critical factor in determining vulnerability to plastic-related health effects, with infants in the womb and young children being particularly sensitive.
Below, we provide an overview of MNP effects on the gut, lung, brain and reproduction. The gut and lung are the first organ systems impacted following exposure to plastics via ingestion or inhalation. The brain, reproductive system, and developing organism have been identified in epidemiologic studies as targets of concern.
NP effects on the gut
Since ingestion is a primary route of exposure to NPs, the GI tract has high exposure to NPs. But what do NPs actually do to the gut? While this is a nascent field, our understanding of the impacts of NPs on gut health is rapidly expanding.
NPs present in food and water come into physical contact with the intestinal wall where they can negatively impact gut function via indirect or direct mechanisms (Figure 2). Indirect mechanisms include NP interference with the ability of the intestine to process essential nutrients, particularly fats. While some studies in animal and cell models observed reduced fat digestion and absorption following exposure to NPs, others reported the opposite. Decreased fat absorption or malabsorption can lead to unhealthy weight loss and digestive issues like diarrhea, whereas increased fat absorption can promote weight gain associated with metabolic dysfunction. Additionally, since dietary fat absorption is required for the uptake of essential fat-soluble vitamins, depression of fat absorption by NPs may result in vitamin deficiencies. Early research suggests that vitamin D absorption may be particularly affected by MNPs. This is especially concerning because vitamin D deficiencies, which affect 1 in 4 adults in the United States, are implicated in a number of chronic disease states.
The intestine is not only the main site of nutrient absorption but also a critical barrier between the outside environment and internal tissues. NP interactions with the intestinal wall can directly injure the epithelial barrier, causing cell damage and inflammation. The resulting compromise of the intestinal barrier integrity, evident as small gaps in the intestinal wall, causes “leaky gut syndrome”, a condition in which harmful substances and bacteria that would otherwise be retained within the lumen of the GI tract and eliminated in the feces are able to readily cross the intestinal wall to enter the body. Once in the systemic circulation, harmful agents are distributed throughout the body where they can trigger inflammation in tissues located at a distance from the gut.
Nanoplastics have also been shown to alter the gut microbiome. The gut microbiome consists of the viruses, bacteria, and other microbes that inhabit the entirety of the GI tract, with the highest concentration being in the large intestine. These microbes support human health, and disruption of the gut microbiome is implicated in cancer, autoimmune disease, metabolic disorders, and chronic diseases in all the major organ systems, including the brain.
NPs can affect the microbiome via multiple mechanisms. First, NPs may alter the environment of the gut, giving survival advantages to certain bacterial species over others. A healthy gut depends on a delicate balance of microbes, and disrupting that balance, which is referred to as gut dysbiosis, can alter microbial diversity in the gut, which is linked to negative health outcomes. For example, microbial dysbiosis increases the chances of colonization of the gut by pathogenic organisms and the entrance of pathogens into the bloodstream through a leaky gut. Bacteria may also be capable of breaking down NPs into smaller particles that can more easily cross the intestinal barrier to gain access to the systemic blood system. While there is evidence that NPs can cause dysbiosis and it is known that gut dysbiosis can lead to wide-ranging health effects, the long-term consequence of NP effects on the gut microbiome on human health has yet to be established.
Once in the GI tract, NPs may also be taken up by immune cells in the gut. The gut contains several types of immune cells that protect against harmful bacteria and allergic reactions to food. However, when these cells encounter NPs, they can react as if they were dealing with a harmful intruder. This causes the immune cells to release signaling molecules that trigger inflammation in the body. Although this phenomenon has not been studied in humans, research in experimental models has established that immune cells in the gut are targets in NP toxicity. For example, in zebrafish, NPs cause gut inflammation, change mucus production, and alter the protective immune cells that line the gut. Similar responses to MNPs have been described in mouse models.
Uptake of NPs by gut macrophages, a type of immune cell that samples intestinal content to identify harmful entities, can damage the internal organelles of the macrophage, resulting in macrophage release of molecules called Danger Associated Molecular Patterns (DAMPs). DAMPs trigger macrophages and other immune cells in the area to release cytokines that cause inflammation. Additionally, specific cell types in the intestine can transport NPs into the lymphatics. Once circulating in the lymph, NPs can modulate signaling pathways and gene expression in diverse cell types throughout the body to alter their function.