George Ordiway, a PhD student in the laboratory of Dr. Jason Tait Sanchez at Northwestern University, discusses how patterned activity in the nervous system permits a wide range of biologically relevant functions, including auditory development
Our thoughts, memories, movements and sensory experiences are the result of electrical activity generated in the brain. Billions of cells make up the brain. These cells, called neurons, communicate with each other by sending electrical signals known as action potentials. Action potential properties are controlled in part by specialised membrane proteins called ion channels, of which there are numerous types. Ion channel expression is incredibly complex, and varies throughout the brain, so neurons are often classified by their functional phenotype, i.e., the pattern of their action potential firing. In this research profile, we briefly consider action potential firing patterns and the functions they serve in both normal and diseased states. We then focus on specialised firing patterns of auditory neurons and their role in hearing.
Diversity of action potential firing patterns
Since the brain is responsible for encoding all sensations and planned body movements, a vast diversity of firing patterns exists. Neurons specialised for hearing act differently than neurons for vision, but even within one sensory modality, variety in firing patterns are ubiquitous1. Ion channels being composed of various multiple subunits is one reason for such diversity.
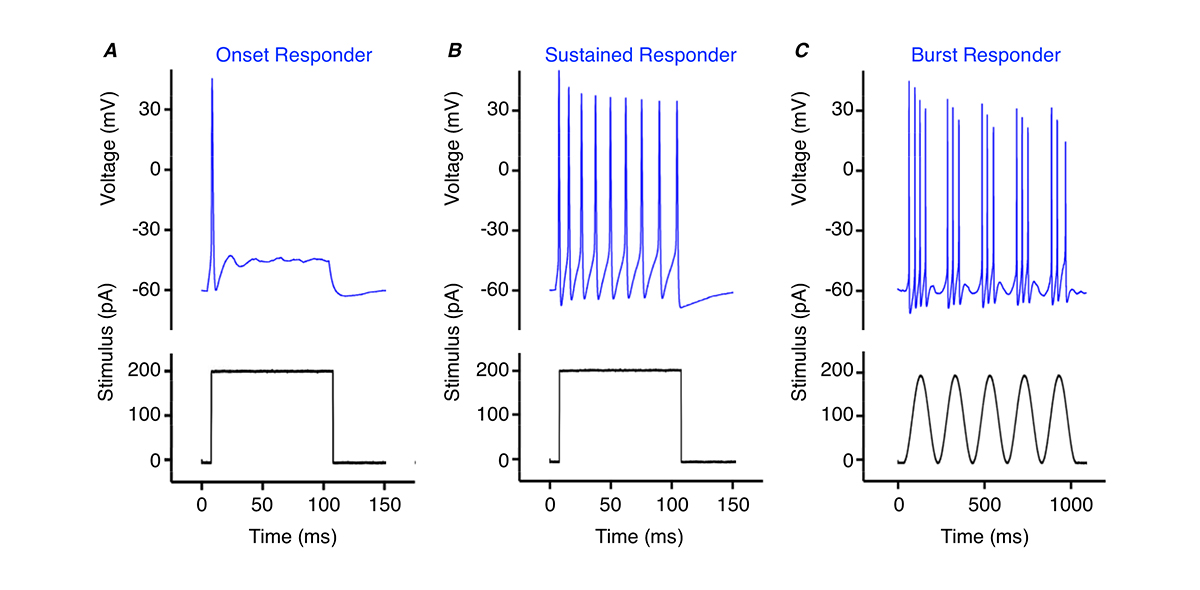
For example, the potassium ion channel has more than 100 subunits2. This is multiplied by the fact that ion channels can be regulated by molecules like glutamate3, dopamine4, nitrous oxide5, cyclic AMP6, and neural growth factors called neurotrophins7, [See Open Access Government January 2019 and April 2019]. While this article will focus on firing patterns in the auditory system, firing patterns in the motor system also play a critical role in biologically relevant functions.
Action potential firing patterns in the motor system
Motor patterns are ubiquitous, from the rhythmic beating of the heart to the flapping of a bird’s wings. Such movements are possible because of the action potential firing patterns of neurons and muscle cells. This patterned activity can be thought of as oscillations8. Oscillation patterns are also seen in spontaneous neuronal activity, a good example being Parkinson’s disease. Parkinson’s is characterised by uncontrollable and spontaneous movement tremors. Recordings of Parkinson’s tremors in monkeys found oscillations at 3-19 cycles per second (Hz).
Remarkably, this oscillating firing pattern is also observed at the individual neuronal level in Parkinsons9, which is critical to its diagonosis and treatment. However, patterned activity in Parkinson’s may be the exception that proves the rule: Patterned activity is usually beneficial – if not necessary – for normal development and brain activity. This is especially true in developing sensory systems like vision and hearing. With vision, patterned activity is needed for eye specific segregation10. Auditory development is also dependent on patterned activity.
Action potential firing patterns in the developing auditory system
An exemplar of auditory development includes research in animal models like mammals and birds. Mice are born deaf, but their auditory pathways are wired to function when the onset of hearing begins. This brings up an intriguing question; how does auditory neural circuitry develop without sensory stimulation? The answer is spontaneous, patterned activity.
For example, supporting cells release ATP to activate hair cells in the cochlea, which leads to bursts of firing activity in auditory spiral ganglion neurons11, 12. This pattern of spontaneous activity, transmitted to the central auditory structures, changes across development. Firing patterns are random at first, gradually change to rhythmic bursts, and then show periods of higher frequency firing, corresponding to before, during and after the onset of hearing, respectively13. Once the auditory system is functionally mature, firing patterns are crucial to encoding different aspects of sound like frequency, intensity and temporal information found in complex signals like speech. Diverse firing patterns are observed in every region of the auditory pathway.
For example, in the cochlear nucleus – a lower auditory brainstem structure in both mammals and birds– as many as six different firing patterns have been well documented and studied with respect to different auditory functions1, 14, 15. Cochlear nucleus neurons receive input from one ear, but their target output neurons are located on both sides of the brainstem. These outputs are responsible for our ability to hear sound from both sides of the head on the order of tens of microseconds; a remarkable biological feat responsible for sound localisation and discriminating complex sounds in background noise16.
Thus, cochlear nucleus neurons are critical for encoding frequency, amplitude and timing aspects of sound. In part, this is due to the firing pattern of action potentials at the onset of a stimulus (onset responders, Fig. 1A), throughout the stimulus (sustained responders, Fig. 1B), or rapid brief periods during the stimulus (burst responders, Fig 1C). Neurons with different firing patterns send outputs to many different auditory regions, critical in processing the numerous features of complex sounds.
One example come from the chicken nucleus magnocellularis – an analogous auditory structure to the mammalian cochlear nucleus. We recently reported the firing pattern from a newly discovered region responsible for processing different frequency elements of sound. These neurons, referred to as NMc, are in stark contrast with other regions where neurons fire a single, precisely timed, onset action potential to stimulation (Fig. 1A). NMc neurons fire multiple bursts of action potentials to slow and gradual stimulation and are hypothesised to respond to ultra-low sound frequencies as low as 2Hz (Fig. 1C) 17, 18. This unique firing pattern suggests a novel biological function related to the processing of sound that differs from the typical pattern of firing responsible for binaural hearing.
Although burst firing in mammalian sensory systems is not uncommon 19-24, it was previously unobserved in the chicken auditory brainstem. This new pattern of firing suggests optimisation for an aspect of sound processing not well understood. Nonetheless, it argues for conserved functions across vertebrates for similar sensory encoding of the surrounding environment.
1 Leao, R.M., The ion channels and synapses responsible for the physiological diversity of mammalian lower brainstem auditory neurons. Hear Res, 2019. 376: p. 33-46.
2 Bean, B.P., The action potential in mammalian central neurons. Nat Rev Neurosci, 2007. 8(6): p. 451-65.
3 Sanchez, J.T., D. Gans, and J.J. Wenstrup, Contribution of NMDA and AMPA receptors to temporal patterning of auditory responses in the inferior colliculus. J Neurosci, 2007. 27(8): p. 1954-63.
4 Ballo, A.W., F. Nadim, and D. Bucher, Dopamine modulation of Ih improves temporal fidelity of spike propagation in an unmyelinated axon. J Neurosci, 2012. 32(15): p. 5106-19.
5 Kopp-Scheinpflug, C., B.M. Pigott, and I.D. Forsythe, Nitric oxide selectively suppresses IH currents mediated by HCN1-containing channels. J Physiol, 2015. 593(7): p. 1685-700.
6 Yin, X.L., et al., Accelerated Development of the First-Order Central Auditory Neurons With Spontaneous Activity. Front Mol Neurosci, 2018. 11: p. 183.
7 Schecterson, L.C., et al., TrkB Downregulation Is Required for Dendrite Retraction in Developing Neurons of Chicken Nucleus Magnocellularis. Journal of Neuroscience, 2012. 32(40): p. 14000-14009.
8 Marder, E. and D. Bucher, Central pattern generators and the control of rhythmic movements. Curr Biol, 2001. 11(23): p. R986-96.
9 Raz, A., E. Vaadia, and H. Bergman, Firing patterns and correlations of spontaneous discharge of pallidal neurons in the normal and the tremulous 1-methyl-4-phenyl-1,2,3,6-tetrahydropyridine vervet model of parkinsonism. J Neurosci, 2000. 20(22): p. 8559-71.
10 Xu, H.P., et al., Retinal Wave Patterns Are Governed by Mutual Excitation among Starburst Amacrine Cells and Drive the Refinement and Maintenance of Visual Circuits. Journal of Neuroscience, 2016. 36(13): p. 3871-3886.
11 Lippe, W.R., Relationship between frequency of spontaneous bursting and tonotopic position in the developing avian auditory system. Brain Research, 1995. 703(1-2): p. 205-213.
12 Tritsch, N.X., et al., The origin of spontaneous activity in the developing auditory system. Nature, 2007. 450(7166): p. 50-5.
13 Tritsch, N.X. and D.E. Bergles, Developmental regulation of spontaneous activity in the Mammalian cochlea. J Neurosci, 2010. 30(4): p. 1539-50.
14 Hong, H., et al., Developmental Profile of Ion Channel Specializations in the Avian Nucleus Magnocellularis. Front Cell Neurosci, 2016. 10: p. 80.
15 Brown, D.H. and R.L. Hyson, Intrinsic physiological properties underlie auditory response diversity in the avian cochlear nucleus. J Neurophysiol, 2019. 121(3): p. 908-927.
16 Carr, C.E. and C. Koppl, Coding interaural time differences at low best frequencies in the barn owl. Journal of Physiology-Paris, 2004. 98(1-3): p. 99-112.
17 Wang, X., et al., Distinct Neural Properties in the Low-Frequency Region of the Chicken Cochlear Nucleus Magnocellularis. eNeuro, 2017. 4(2).
18 Hong, H., et al., Diverse Intrinsic Properties Shape Functional Phenotype of Low-Frequency Neurons in the Auditory Brainstem. Front Cell Neurosci, 2018. 12: p. 175.
19 Zeldenrust, F., W.J. Wadman, and B. Englitz, Neural Coding With Bursts-Current State and Future Perspectives. Front Comput Neurosci, 2018. 12: p. 48.
20 Samengo, I. and M.A. Montemurro, Conversion of phase information into a spike-count code by bursting neurons. PLoS One, 2010. 5(3): p. e9669.
21 Eyherabide, H.G., et al., Bursts generate a non-reducible spike-pattern code. Front Neurosci, 2009. 3(1): p. 8-14.
22 Lisman, J.E., Bursts as a unit of neural information: Making unreliable synapses reliable. Trends in Neurosciences, 1997. 20(1): p. 38-43.
23 Krahe, R. and F. Gabbiani, Burst firing in sensory systems. Nat Rev Neurosci, 2004. 5(1): p. 13-23.
24 Chan, H.K., et al., Burst Firing Enhances Neural Output Correlation. Front Comput Neurosci, 2016. 10: p. 42.
Please note: This is a commercial profile