Professor Friedemann Freund, SETI Institute/NASA Ames Research Center, provides an insight article into the complexities of oxygen
We breathe oxygen, O2. We use it to power the molecular engines in our bodies that keep us up and running. As part of this process O2 molecules are broken up. Electrons are taken from the carbon and hydrogen in organic compounds. They are transferred onto oxygen atoms that change their valence from zero to minus two, forming carbon dioxide and water. Simplifying the organic matter as –CH2– we can describe this process by writing:
–CH2– + 1½ O2 => CO2 + H2O
However, biologists and the medical community have long known that there are intermediate stages with oxygen becoming indiscriminately aggressive in its chemical and biochemical reactivity. The reason is simple: Nature tends to transfer single electrons from one molecule to the next or from one atom to the next – never two at a time. This means that, when oxygen atoms participate in chemical reactions in our bodies, they pass through the O- stage, a radical. O- can create havoc in living cells. They have earned the moniker “Reactive Oxygen Species” or ROS for short.
To counteract the detrimental effects of ROS Life relies on enzymes such as superoxide dismutase (SOD) and catalase. They contain iron that can switch back and forth between divalent (Fe2+) and trivalent (Fe3+), helping to render ROS less harmful.
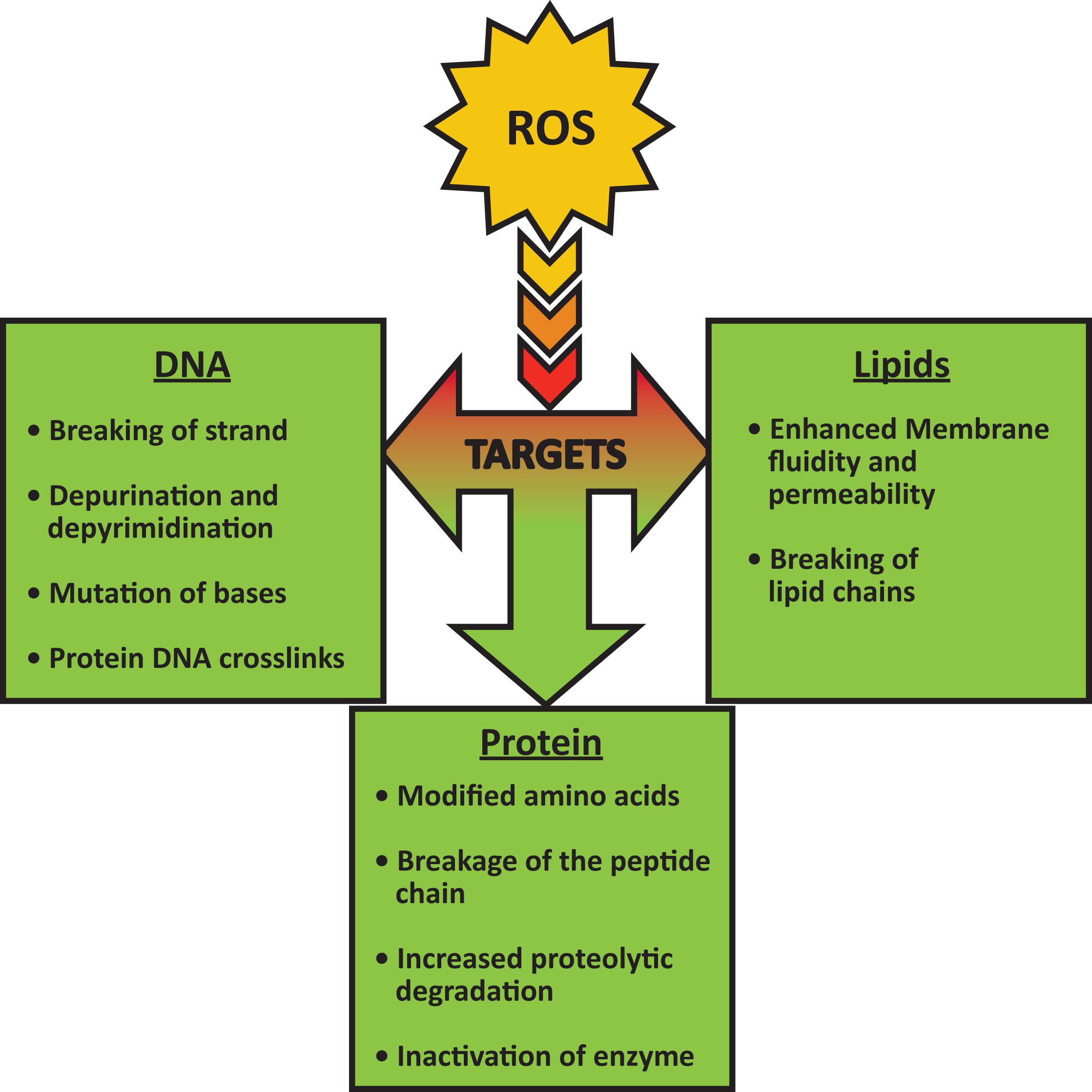
Figure from: Reactive Oxygen Species (ROS) and response of antioxidants as ROS-scavengers during environmental stress in plants. Kausik Das, Aryadeep Roychoudhury. Published in Front. Environ. Sci. 2014. DOI:10.3389/fenvs.2014.00053
There is a widespread belief within the biochemical and medical communities that ROS first appeared in Earth’s history after Life “invented” oxygenic photosynthesis to produce O2. The underlying reasoning has a logic ring: ROS are a byproduct of biochemical reactions in oxygen-breathing organisms. However, the idea that the appearance of ROS is tied to the advent of oxygenic photosynthesis stands in stark contrast to the observation that the oldest single cell organisms produce enzymes such as SOD and catalase.
These microorganisms live in strictly anaerobic environments and must have done so ever since they appeared on Earth. The question therefore arises: Why did early microorganisms, whose origin predates oxygenic photosynthesis by at least a billion years, produce SOD and similar antioxidant enzymes, if there were no ROS-like oxidants around in their environments?
Could it be that the geological environment produces ROS-like oxidants and has done so since the beginning of Life?
According to textbook knowledge the answer is “no”. Rocks in Earth’s crust consist of minerals, predominantly silicates, in which oxygen always occurs in the 2- valence state. This concurs with the fact that the Earth beneath our feet retains the memory of its origin as a planet accreting in the highly reducing, hydrogen-dominated solar disk some 4.5 billion years ago. Because the 2- valence state of oxygen is so prevalent, the idea became firmly rooted in the minds of most geoscientists that oxygen in the geological environment is always O2-.
However, there is a process, thermodynamically fully certified, by which oxygen in the valence state 1- is introduced into rocks in a sneaky way. It begins with the crystallization of minerals from magmas that are always laden with fluid phase components, often mostly water, H2O.
Thermodynamics mandates that, whenever a mineral crystallizes in an H2O-laden environment, small but non-zero quantities of H2O will enter the crystalline matrix in the form of hydroxyls, typically O3Si-OH. In other words, hydroxyls become “impurities”, even in minerals that have no place for them in their crystal structure.
Thermodynamics further mandates that, upon cooling, impurity concentrations must decease. This can only be done by hydroxyls diffusing towards the grain boundaries. At the same time diffusion slows down. When diffusion comes to a halt, typically around 500°C, a new reaction takes place that mainstream geoscience has failed to notice: Pairs of hydroxyls undergo a redox conversion and turn into peroxy plus molecular hydrogen:
O3Si-OH HO-SiO3 O3Si-OO-SiO3 + H2
Redox conversions are common in chemistry, ratcheting up the valence of one and down the valence of the other. In the case considered here, hydroxyl oxygens change from 2- to 1-, while hydroxyl protons change from 1+ to 0. The two O- form a peroxy bond. As long as they are held together, these O- are chemically inactive. This changes when the O-–O- bond breaks.
To understand this step requires a bit of semiconductor physics. The reason is that, when the O-–O- bond breaks, electronic charge carriers are generated, electrons e’ and holes h•, similar to the electrons and holes in transistors that run our electronics. In minerals and rocks, the e’ and h• behave differently than in archetypical semiconductors: the e’ get stuck in the broken peroxy bonds, while the h• have the remarkable ability to leave the sites, where they were born. They can spread into the surrounding rocks, traveling far afield, easily tens of kilometres and more.
Why is this important? Because those h• are defect electrons in the matrix of O2-, chemically O-. They are ROS – Reactive Oxygen Species. They loom everywhere in the geological environment and have done so since the Earth formed as a rocky planet some 4.5 billion years ago. Therefore, when Life appeared on Earth, the first organisms already had to content with peroxy and ROS. This is why they needed enzymes and why SOD and catalase are found in the genetically oldest lifeforms on Earth dating back at least a billion years before the advent of oxygenic photosynthesis. They needed antioxidant enzymes to survive in an early Earth environment that contained peroxy and could unleash viciously oxidizing ROS.
Please note: This is a commercial profile