F Barry Dunning, a professor in the Department of Physics & Astronomy at Rice University, explores the atomic states within Rydberg atoms and their possibilities in atomic engineering
One overarching goal in the present quantum revolution is the development of techniques to manipulate atomic states and create “designer” atoms with carefully-tailored properties for application in areas such as quantum sensing and quantum computation. Atoms in highly-excited “Rydberg” states provide a powerful platform for exploring and testing different possible protocols for such “atomic engineering” and have also furnished new insights into light-matter interactions and the non-linear dynamics of driven systems.
As discussed in a recent e-book, if an electron in an atom is given additional energy, it can transition to one of an allowed series of excited states whose characteristics depend on the principal quantum number n. In particular, atomic radii grow rapidly with n, scaling as n2, and by n ~ 1000 approach 0.1 millimeters. Given their large physical size, many properties of such atoms can be discussed using the classical Bohr model of the hydrogen atom, namely a point-like electron moving in orbit about the nucleus held in place by its electrostatic attraction to the nucleus.
“Kicked” Rydberg atoms
For large values of n the electric field the electron experiences from the nucleus becomes very small, allowing its motion to be controlled by the application of even small external electric fields in the form of microwave radiation or pulses with durations less than the electron orbital period, which increases as n3 and reaches ~ 150 nanoseconds at n ~ 1000. For sufficiently short pulses, each one simply delivers an impulsive “kick” to the electron, whereupon a carefully-tailored sequence of such kicks can be used to control its motion and drive it into a desired final state much as the orbit of a satellite can be manipulated by a series of carefully-timed rocket bursts.
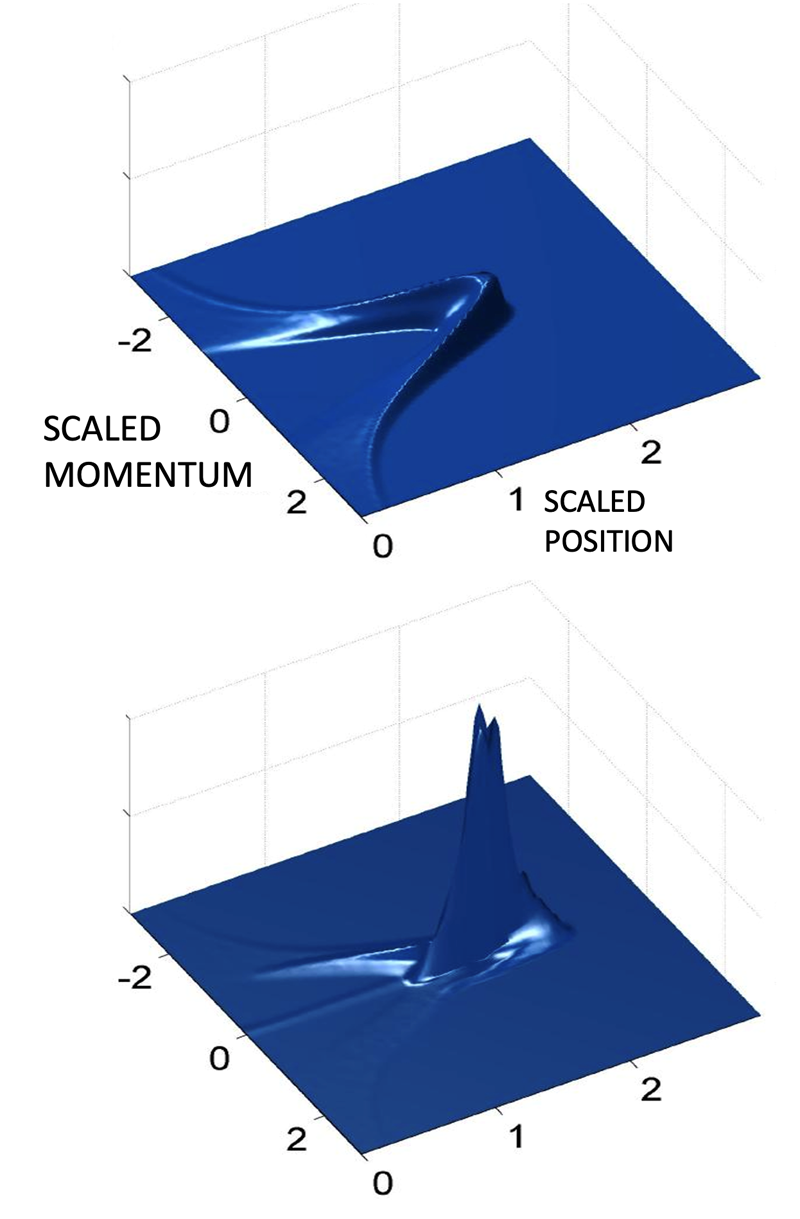
Initial studies of “kicked” Rydberg atoms utilized quasi-one-dimensional (quasi-1D) atoms produced by laser excitation in a weak DC electric field, in which the electron executes a highly elliptical orbit whose axis is aligned with the field. It might be expected that were such atoms to be subjected to a series of strong equally-spaced kicks directed along this axis, the electron would simply be kicked off the atom. Such behaviour was indeed seen but it was observed that for certain kick strengths and frequencies, the atoms could survive many, many kicks. Such “dynamical stabilization” was surprising and led to the realization that “islands of stability” could form within an otherwise chaotic sea. Their analysis provided new insights into both classical and quantum chaos and the relationship between them.
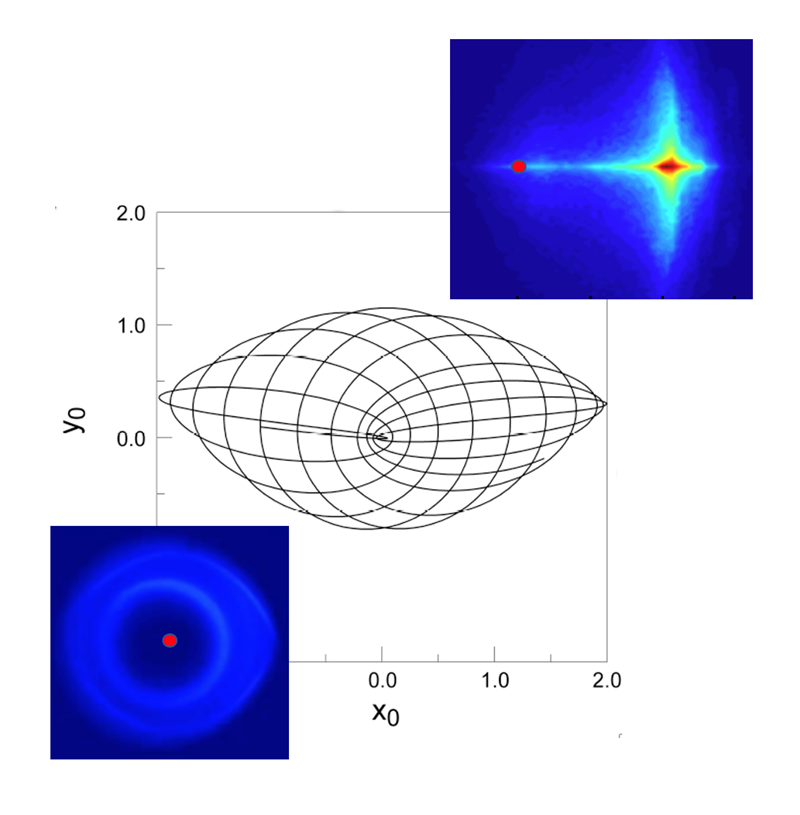
In the initial quasi-1D atoms, the electron probability density, characterized by its wave function, is distributed around its orbit. Application of a single kick, however, can lead to its transient localization in the outermost regions of the orbit, thereby providing a well-defined starting point for further atomic engineering. In particular, the electron motion can then be locked to an external microwave drive field or a periodic series of unidirectional kicks. This allows the electron to be transported to states of higher or lower n simply by slowly varying the frequency of the drive field. Alternately, the application of a sideways kick at the time of optimum localization can launch the electron into a near-circular orbit and create a quasi-two-dimensional “circular state.”
Circular states which mimic a tiny solar system
Circular states can also be generated by suddenly applying smaller, somewhat longer transverse pulsed electric fields to a quasi-1D atom. This field causes the electron orbit to process, resulting in periodic transitions between highly-elliptical and near-circular paths. If the field is turned off just as circularity is reached, the electron remains frozen in a circular orbit. Following turn-off, the electron wavefunction becomes transiently localized within its orbit. However, it does not remain localized and spreads around its orbit resulting in so-called “dephasing.” Classically, such dephasing is irreversible. In contrast, quantum mechanics predicts that because atomic energy levels are quantized, the wavefunction will periodically relocalize, leading to “quantum revivals.” Such revivals are indeed observed, indicating that even at high n, where classical physics might be expected to rule, quantum mechanical effects can still be observed. Delocalization can be suppressed by application of a carefully-tailored periodic sequence of drive pulses or a microwave field, allowing the creation of atoms whose behaviour mimics that of the Bohr atom, or of a tiny solar system.
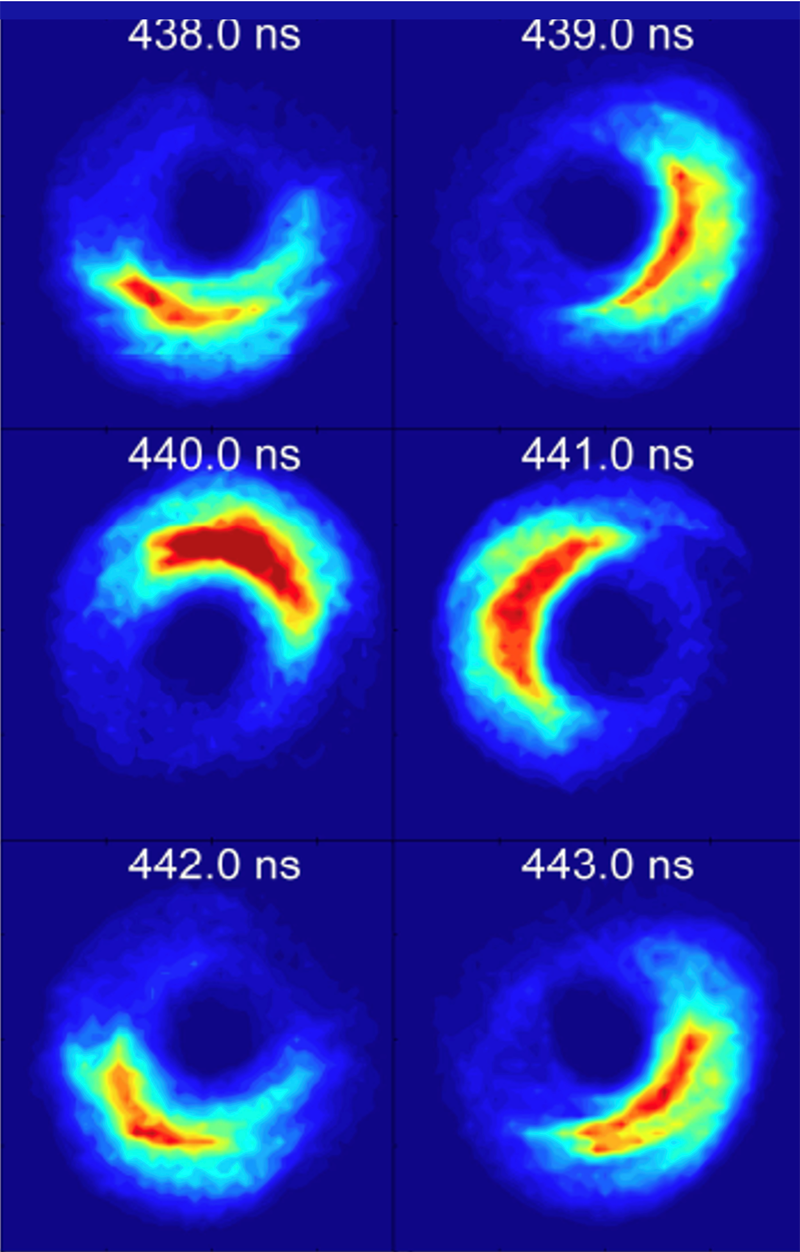
Perturbations introduced by the atomic environment can lead to “decoherence” which results in a transition from quantum to classical behaviour and represents a serious problem to the operation of quantum devices. High-n Rydberg atoms furnish a sensitive probe of environmental influences, permitting, for example, detailed studies of decoherence induced by stray background electrical fields whose “noise” characteristics can be carefully-controlled. Nonetheless, this work also demonstrated that under appropriate conditions, quantum information can be imprinted, stored, and read out in Rydberg atoms.
Illuminating the behaviour of other mesoscale systems
Insights gained through Rydberg atom engineering are of value in many areas. For example, they illuminate the behaviour of other mesoscale systems, such as quantum dots, that also play a prominent role in exploring concepts and technologies related to quantum information processing. Furthermore, since frequencies of a few gigahertz and fields of a few volts per centimetre appear ultrafast and ultra-intense to a high-n atom, Rydberg atoms also speak to the engineering of low-lying atomic (and molecular) states at the sub-nanoscale using intense ultrashort (attosecond) laser pulses. In addition, Rydberg atoms provide a valuable bridge between the micro- and macroscopic worlds and help elucidate the transition from quantum to classical behaviour.
This is the final article in a series authored by Dr. F. Barry Dunning discussing the properties and applications of Rydberg atoms. If you would like to read his earlier articles, follow these links:
- Giant Rydberg atoms: From scientific curiosity to quantum sensors
- Rydberg atoms: quantum electric field sensors
-
Rydberg atoms and optical tweezers: new opportunities in quantum science